Infrared Spectroscopy and Its Use in Gemology
ABSTRACT
Since its discovery at the beginning of the nineteenth century, infrared light has proven to be a versatile tool for scientists, industrialists, and consumers. The gem industry has benefited from the power of infrared spectroscopy in gemstone identification for more than 30 years. By measuring the frequencies of vibrations of bonds between atoms in the structure of a gemstone, scientists can determine the composition and atomic arrangement of many important defects that indicate whether a gem is natural, laboratory-grown, or treated. Clever adaptations of instrument hardware and sampling techniques have allowed for efficient and accurate analysis of diamonds and many of the most important colored gems such as ruby, sapphire, emerald, and jadeite. No gemologist’s toolbox is complete without an understanding of the power of infrared spectroscopy for gem identification.
The foundations of gemology lie in visual observation and mineral properties. Many basic gemological characteristics including refractive index, specific gravity, pleochroism, fluorescence, optic figure, and color zoning are produced by the specific atomic structure of the gem being examined. Spectroscopy is the tool of choice in gemology for examining atomic structure due to its nondestructive nature and sensitivity. Absorption spectroscopy (measuring the light that is absorbed by a gem) was among the earliest techniques used to evaluate atomic level imperfections (or defects) in gemstones. Depending on the type of absorption spectroscopy used, a laboratory can evaluate the basic identity of a gem, indications of treatment, or the cause of its color. Visible absorption is effective for color investigations and is the subject of another article in this issue of Gems & Gemology (Jin et al., 2024). This article focuses on infrared (IR) absorption spectroscopy, a very important tool that measures atomic vibrations to evaluate atomic structure and identify gems and treatments (e.g., Fritsch and Stockton, 1987; Lowry, 2008; Breeding et al., 2010).
In order to understand infrared absorption spectroscopy, a brief discussion of the electromagnetic spectrum is required. In simple terms, the electromagnetic spectrum encompasses all radiation (or “light”) in order of increasing frequency/energy and decreasing wavelength: radio waves, microwaves, infrared, visible, ultraviolet, X-rays, and gamma rays (Jenkins and White, 1976; Nassau, 2001) (figure 1). The IR region occurs at slightly lower energies (and longer wavelengths) than the red end of the visible spectrum and is invisible to the human eye. It is used in many heating, imaging, and communication devices, some as simple as television remote controls and other home appliances. The IR region is subdivided by wavelength into near (~0.75–5 μm), mid (~5–30 μm), and far (~30–1000 μm). Most scientists, however, prefer to use wavenumbers (units of 1/cm or cm–1) to describe IR light because the numbers are manageable and linearly correlated with the vibrational energy being measured. It is important to remember that as wavenumbers and the corresponding energy increase, the wavelength decreases. The mid-IR range (~2000–333 cm–1) is most useful in gemology, but some important features also occur in the near-IR (~13333–2000 cm–1).
IR light was first discovered in 1800 by British astronomer Sir William Herschel, using thermometers to measure heat beyond the red part of the visible spectrum (figure 2, left). That same discovery is the underpinning of today’s IR thermal imaging devices. The first generation of IR instruments became commercially available in the late 1950s. These instruments were dispersive IR devices (prism or grating monochromators) that required each wavelength of light to be measured individually, making them very slow and expensive (figure 2, right).
IR spectroscopy as we know it today is almost exclusively performed using the Fourier-transform infrared (FTIR) technique. With the invention of the Michelson interferometer in 1881, it was possible to measure many IR wavelengths at once, but the mathematical transformation of the interferometer data required hours of work on very expensive computers. The first FTIR spectrum was collected in 1949, before dispersive IR instruments became available, but it was not until 1966 that a Fourier-transform algorithm was discovered, which allowed for quick processing and conversion of data to make FTIR viable. The 1970s saw the first commercial FTIR devices, and by the 1990s, FTIR had become mainstream (Becker and Farrar, 1972; Sun, 2009; Smith, 2011).
Gemological laboratories regularly use FTIR for gemstone analysis, and GIA has done so since 1986 (Fritsch and Stockton, 1987). This bulk analysis technique has proven effective for various applications related to diamond and colored stone identification and treatment detection that will be discussed later in this article. It not only allows measurement of subtle details of the atomic structure of gems, such as the presence of hydrogen species in sapphire or individual nitrogen impurity atoms in diamond, but also detects materials that have been mechanically introduced into microscale pores or cracks in gemstones to improve stability and/or clarity (e.g., jadeite, turquoise, and emerald) (Fritsch and Stockton, 1987; Breeding and Shigley, 2009; Thongnopkun et al., 2022). The goal of this article is to introduce readers to the basic concepts of FTIR analysis of gemstones and some of the major applications for which it is currently used. It is not intended as a comprehensive review of IR analysis but as a tool to help readers better understand FTIR and its importance to the gem trade.
INSTRUMENTATION AND DATA COLLECTION
FTIR instruments have been widely commercially available since the 1990s. They range from desktop devices to handheld units and low-resolution, application-specific devices to very expensive, multifunctional research-grade instruments. Well-established manufacturers include Agilent, Bruker, Jasco, Perkin Elmer, Shimadzu, and Thermo Fisher (figure 3). More recently, several smaller companies have also entered the market. While the uses and analytical capabilities of these instruments vary depending on available features and design, all FTIR spectrometers contain the same basic components: IR source, aperture, interferometer (including beam splitter and mirrors), sample introduction system, detector, and processing computer.
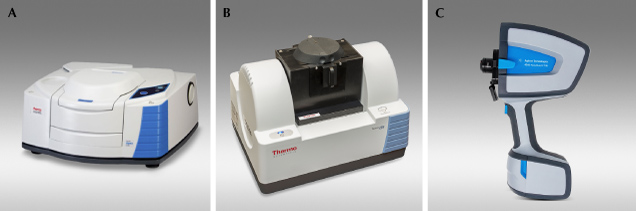
The basic principle of FTIR involves a source producing IR light that is directed through an aperture (or controlled opening) into a complex lens called a beam splitter that splits the light into two beams, one of which strikes a fixed-position mirror and the other a moving mirror. The two beams are reflected back into the beam splitter, where they are recombined and directed through the sample to a detector (Smith, 2011) (figure 4). The difference in the distance the light travels from each of the two differently positioned mirrors produces a range of constructive and destructive interference of the light waves as the moving mirror travels a designated distance (its position is monitored by a tracking laser at all times). The continuous range of travel of the moving mirror allows for simultaneous collection of the complete spectrum. The atomic structure of the gemstone sample selectively absorbs or attenuates certain wavelengths of IR light. The remaining light hits the detector and is converted into an electronic signal called an interferogram that is mathematically transformed (Fourier transform) as a function of time into an FTIR spectrum (Sun, 2009; Smith, 2011). While the concept sounds complicated, the end result is a graph that shows which IR wavelengths are more strongly and weakly absorbed by the atomic bonds in the gemstone. Gemologists and scientists compare this spectrum to known reference spectra to evaluate the identity of a gem, its impurities, or the presence of treatment.
Gemologists primarily rely on the mid- and near-IR regions, which are more easily measured and contain the information most readily applied to gemstone analysis. The instrument components themselves dictate which of these regions can be measured, as well as the data detail and quality that can be obtained. To collect mid-IR range data, for example, a ceramic IR source (silicon carbide rod electrically heated to >1000°C) is optimal, while a tungsten-halogen lamp is preferable for near-IR. Likewise, different beam splitters are more efficient in different IR regions. For mid-IR, a potassium bromide (KBr) beam splitter is used, while a quartz beam splitter works more efficiently for near-IR. The aperture and interferometer mirror travel distance together determine the resolution of an FTIR spectrum. Resolution refers to a spectrometer’s ability to differentiate closely spaced spectral features. Typical FTIR resolutions include 16, 8, 4, 2, or 1 wavenumber (or cm–1) (figure 5). For example, an FTIR resolution of 4 cm–1 means that only features separated by four or more wavenumbers can be separately identified. For most colored gemstones, FTIR features are broad enough that 4 cm–1 resolution is adequate. Some diamond defects, however, require 1 cm–1 to resolve. The FTIR detector also plays an important role in the data acquired. The two main detectors are mercury cadmium telluride (MCT) and deuterated triglycine sulfate (DTGS). MCT detectors are up to 10× more sensitive and faster, but they often require cooling by liquid nitrogen and are more expensive. DTGS detectors are less expensive and still adequate for many applications (Sun, 2009; Smith, 2011).
A few additional details warrant discussion with FTIR: sample temperature, signal-to-noise ratio, purging, and orientation/polarization. FTIR spectra are collected for all gemstones at room temperature, as there is no distinct advantage in cooling the sample in the IR region. The signal-to-noise ratio is an indicator of how distinguishable small absorptions in the spectrum are from the background noise level (figure 6). The signal-to-noise ratio can be improved with MCT detectors and increasing the number of scans to be averaged for the final spectrum. Other aspects of the Fourier transform, such as the apodization function and zero filling (mathematical functions applied to the data during the transform), will also affect the shape of peaks and the appearance of the spectrum but are beyond the scope of this discussion. To avoid atmospheric contaminants (water vapor and carbon dioxide [CO2] gas) from being measured in an FTIR spectrum, many instruments allow for purging. This procedure involves the continuous cycling of dry air or inert gas (usually nitrogen) through the instrument interior and sample chamber to force out any contaminated air. Finally, IR absorptions are often affected by the crystallographic orientation of a gemstone. While not a common practice in gemology, some mineralogical research requires polarization of the IR beam in order to measure the orientation dependence of features in the spectrum.
One of the most important components of an FTIR instrument is the sample introduction system. Gemstones are faceted to control the movement of light through the stone and to enhance the color or brilliance (figure 7A). The goal of faceting is often counter to the needs of spectroscopy, making it somewhat difficult to obtain good-quality FTIR spectra from gemstones. In gemology, there are three main methods of FTIR sample measurement: transmission, reflection, and diffused reflection (or DRIFTS—diffused reflectance infrared Fourier-transform spectroscopy) (Fritsch and Stockton, 1987; Breeding and Shigley, 2009; Thongnopkun et al., 2022). Another method called attenuated total reflection (ATR), which involves direct physical contact with a sample, is widely used for other industrial applications but has only recently been applied in gemology. This technique does have some potential for the analysis of opaque gemstones that have previously required preparation with KBr, as discussed later in this paper with regard to turquoise and amber.
Transmission FTIR is a basic method of passing the infrared beam directly through a sample and examining the transmitted IR beam to measure absorption. A beam condenser sample stage is often used in transmission FTIR instruments to focus the IR beam to a spot and amplify it using carefully aligned mirrors. The stone is placed at the focal point and the IR beam samples all of the stone area through which it passes, providing a bulk averaged absorption spectrum (figure 7B). This method can be difficult for many faceted gems, as direct paths through them are rare. With a carefully constrained beam path length, FTIR data can be quantified using this method.
Reflection FTIR involves reflection of an IR beam off of a flat gem surface (figure 7C). A simple reflection accessory is added to the sample chamber, allowing a gemstone to be placed flat side down on a window. The beam typically does not penetrate below the surface of the gem, and the resultant spectrum is usually different from that measured by transmission. Reflection analysis is very useful for gem identification and for opaque gemstones that cannot be measured by transmission FTIR (Hainschwang and Notari, 2008).
The final technique, DRIFTS, was designed to analyze powders or granular samples by producing multiple internal scattered reflections between the grains. Innovative gemologists, however, modified the technique to allow for efficient FTIR measurements on gems (figure 7D). A faceted stone is placed flat side down on a reflective stage inside a DRIFTS chamber made of multiple mirrors. The mirrors direct the IR beam at a high angle into the side of the gem, causing it to reflect around many times internally and exit through the opposite side at an angle aligned with the mirrors. This clever adaptation allows for bulk sampling of a faceted gem without a straight path through it. The technique may be better described as partial internal reflection, but the resultant FTIR spectrum is identical to a traditional transmission FTIR result.
While nearly all gemological laboratories utilize desktop FTIR instruments, some portable versions are commercially available. These portable FTIRs utilize ATR and reflection FTIR methods to allow for direct sampling of various materials by making physical contact with the instrument’s sample port. As mentioned above, ATR is generally not useful for gem analysis except as a replacement in special sample preparation situations involving destruction of parts of the sample. The reflection FTIR spectra obtained from handheld devices tend to be of lower quality and less useful for gemstone analysis due to the limitations of the detectors (DTGS) and other components required to make the instruments portable.
Recently, a new mid-IR spectrometer using upconversion technology has been proposed as an alternative to FTIR. This instrument could provide an opportunity for faster data acquisition in the mid-IR range (Wang and Takahashi, 2023).
ANATOMY OF AN FTIR SPECTRUM
FTIR spectra reveal absorption (or reflection) peaks that represent variations in the atomic structure of a gemstone, such as impurities or atomic lattice disruptions due to atoms simply being out of place. For the most part, IR energy is absorbed by the bonds between atoms or within molecules, causing them to vibrate in distinct and characteristic ways (Becker and Farrar, 1972; Sun, 2009; Smith, 2011). These vibrations are called modes, and each has a frequency that is unique to the particular bonds and atoms within a material (figure 8). Modes may be symmetric or antisymmetric and comprise movements such as stretching, bending, rocking, wagging, and twisting. Sometimes the same bond may have multiple modes that show up simultaneously in an FTIR spectrum as different absorption peaks at different wavenumbers.
An FTIR spectrum is a graph of data with wavenumber units (cm–1) along the horizontal axis representing the range of IR frequencies being measured and units of transmittance, absorbance, or reflectance on the vertical axis (figure 9). FTIR instruments usually make primary measurements as transmittance (usually given as % of total), but those are mathematically converted (A = –log T, where A is absorbance and T is transmittance) into qualitative absorbance (reported as absorbance units or a.u.) if the IR beam path length is unknown. Quantitative absorption coefficient (units of cm–1) is reported if path length has been accounted for (α = 2.303A/d, where α is absorption coefficient, A is absorbance, and d is the path length typically in units of cm) (Becker and Farrar, 1972; Fritsch and Stockton, 1987; Breeding and Shigley, 2009; Sun, 2009; Smith, 2011). Reflection is typically reported as reflectance (unitless or as % of total).
In situations where the IR beam path length through a sample is known and absorption coefficients are calculated, the heights of individual peaks in the spectrum represent the concentration of particular defects. If appropriate reference gems with known concentrations of defects or impurities are available for comparison, FTIR spectra can yield quantitative atomic compositional data. For diamond, which has intrinsic absorptions in the mid-IR range, FTIR spectra can be normalized and atomic defect concentrations calculated even when the path length is not measurable (Boyd et al., 1994, 1995; Breeding and Shigley, 2009) (figure 10). While normalization introduces some error, the ability to evaluate chemical composition from a diamond’s FTIR spectrum is invaluable to research efforts.
Interest in better understanding the spatial distribution of FTIR features sparked the development of instruments capable of rapidly collecting FTIR data in two or even three dimensions across a sample. FTIR mapping is used in gemological research and involves the acquisition of thousands of individual spectra from each point in a map, with the sample moved by a motorized stage (Howell et al., 2019). The time required for mapping can be significant, depending on the user’s sample size and spatial resolution needs, but the technique uses the same acquisition parameters defined above. A more recently developed technique is FTIR imaging, which is much faster because it employs a focal plane array (FPA) detector, where a series of arranged detectors are effectively used as pixels in an IR camera, allowing a defined sample area to be mapped almost simultaneously.
In addition to absorption peaks from features within a gemstone, a few other aspects of FTIR spectra are important to understand. Surface or atmospheric contamination can show up very prominently in a spectrum and, at times, be difficult to differentiate from actual components of a gem. CO2 occurs as broad, rounded double peaks at ~2200–2400 cm–1 in FTIR spectra (figure 11). Water vapor occurs as numerous narrow absorptions concentrated in three regions of the mid-IR: ~1400–1800, 3500–4000, and 5100–5500 cm–1 (Smal et al., 2014). CO2 and water vapor are the most common atmospheric contaminants in FTIR spectra. CO2 in particular is known to occur in fluid inclusions, and great care must be taken with spectra collection and purging to distinguish gaseous inclusions from contamination. Hydrocarbon surface contamination from finger oils or other organics is also readily observed as three distinctive and broad absorption features between 2830 and 3000 cm–1 (figure 11).
Another potentially confusing feature in FTIR spectra is usually termed “saturation.” FTIR actually measures the IR light transmitted through a stone, and this information is then converted to absorbance, which is effectively the opposite of transmission. When transmission at particular wavenumbers is too low for the instrument detector to measure (meaning almost all light is absorbed by the gem), the resultant absorbance FTIR spectrum often shows irregular areas of very high noise where the detector has become “saturated” (figure 12). The term is misleading in that these are actually areas of little to no signal, but because we convert to absorbance, the data region is at the top of the limit, implying saturation. The data points in these areas are a byproduct of the instrument’s inability to properly represent the data and are thus meaningless other than to indicate very strong absorptions. These areas in an FTIR spectrum should be truncated to avoid confusion and misinterpretation of the data.
MAJOR APPLICATIONS IN GEMOLOGY
FTIR spectroscopy is one of the most common analytical techniques in modern gemology (Fritsch and Stockton, 1987; Breeding and Shigley, 2009; Thongnopkun et al., 2022). Its ability to measure and identify a wide range of gemstones and their treatments at room temperature is particularly valuable for gemologists. Mid-IR spectroscopy is used most often in gemology, and most of the relevant identification features occur in that region. Very little sample preparation is required, and the analysis is rapid and usually provides distinctive results. Its use is most widespread for diamond analysis, as it provides an abundance of information about impurities and their atomic configurations. While IR-related defects in diamonds have been extensively studied and documented, FTIR is also a major analytical tool for ruby, sapphire, emerald, jade, turquoise, amber, and many others. We will review some of the most prominent modern uses of FTIR in gemology below.
At this point, it is helpful to briefly elaborate on the terminology used by scientists and gemologists to describe atomic structures and substitutional elements in minerals. In general, a mineral is considered pure if all of its atoms are in perfect crystallographic positions and no foreign elements have substituted for the primary elements (i.e., diamond consisting of only carbon atoms in perfect tetrahedral arrangement). In nature (and in most laboratory crystal growth), some minor or trace amount of a non-native element is nearly always incorporated during mineral growth or treatment. Likewise, some form of deformation, from an environmental condition such as radiation or heating or simply the incorporation of foreign elements of differing atomic sizes or electric charges during growth, can modify the structural alignment or symmetry of the atoms. These deviations from a perfect structure are often termed “defects” or, in the case of foreign elements, “impurities.” While these terms have a seemingly negative connotation, defects and impurities are actually the subtle components of a gem that often contribute to its beauty, rarity, and value. For example, minor amounts of chromium in corundum create the red color that makes it a ruby, while a few boron atoms among billions of carbon atoms in diamond produced the stunning blue color of the world-famous Hope diamond.
Diamonds. All diamonds (both natural and laboratory-grown) have a distinctive series of intrinsic absorptions in the multiphonon region of the mid-IR, produced by the vibration of covalently bonded carbon atoms in response to IR energy (Anderson, 1943, 1963; Blackwell and Sutherland, 1949; Davies, 1977; Breeding and Shigley, 2009) (figure 13). Phonons are essentially vibrations of groups of atoms in a crystal lattice. Diamond has three distinctive phonon regions in the IR. The intrinsic absorptions from carbon atoms in diamond comprise the two- and three-phonon IR regions. The one-phonon region has no absorption for completely pure diamond (Wilks and Wilks, 1991; Kiflawi et al., 1994). Diamond usually contains nitrogen or boron as impurities (Kaiser and Bond, 1959). Fortunately for gemologists, nitrogen impurities (single, paired, and four N atoms + a vacancy) have distinct absorption features in the one-phonon IR region, while boron (occurring as single atoms only) has distinct peaks across all of diamond’s IR phonon regions. These clear features allow diamond to be classified into distinct “types” such as type Ia, Ib, IIa, or IIb (figure 13). For more detailed information about diamond types and their significance in gemology, see Breeding and Shigley (2009). Understanding diamond type is critical for separating laboratory-grown from natural diamonds. Nearly all colorless laboratory-grown diamonds are type IIa, compared to less than 2% of natural diamonds. Additional IR features such as amber centers (produced by plastic deformation in the earth) and particular types of hydrogen-related peaks are unique to natural or laboratory-grown diamonds and provide further help in identification.
FTIR spectra also provide important details of diamond treatment. Irradiation treatment using electron beams creates atomic vacancies and turns diamonds blue or green colors (or yellow or orange or pink with subsequent heating). This produces IR-active atomic defects that can be seen in FTIR spectra, including H1a (1450 cm–1), H1b (4935 cm–1), and H1c (5165 cm–1). These complex interstitial defects are common in irradiation-treated diamonds and much rarer in naturally colored diamonds (Woods, 1984; Collins, 2001; Liggins et al., 2010) (figure 14). High-pressure, high-temperature (HPHT) treatment can be used to either decolorize brown diamonds to make them near-colorless or create substantial color by rearranging existing atomic defects. For all practical purposes, only type IIa brown diamonds (containing few to no nitrogen impurities) can be decolorized, making the IR spectrum and diamond type determination an important tool. Other IR features including a broad 1480 cm–1 band and combinations of isolated and aggregated nitrogen defects often indicate HPHT treatment in colored diamonds (Collins et al., 2000). HPHT treatment is also known to reduce the height of platelet peaks (planar bunches of interstitial carbon atoms created as nitrogen aggregates; Woods, 1986; see also figure 15) and destroy plastic deformation features such as amber centers (Massi et al., 2005). While most of these IR peaks can be observed in untreated natural diamonds, they are far more prevalent in treated diamonds. By understanding their occurrence in combination with other defects measured through various types of spectroscopy, gemologists can assess whether a diamond is natural, laboratory-grown, or treated in some way to improve its color.
Ruby and Sapphire. Although ruby and sapphire are gem varieties of the mineral corundum and among the highest-value colored gemstones, not all of them come out of the ground with ideal color. Through extensive experimentation, gem treaters and scientists have learned to improve the color of corundum. Heat treatment (or thermal enhancement) has been used for decades to create a more appealing color in lower-value stones. FTIR analysis has proven to be particularly useful in identifying these treatments. For example, many blue sapphires formed in metamorphic environments that have been heat treated to 1500°C to enhance their color will show IR absorptions related to OH stretching modes of water in the corundum structure at 3309, 3232, and/or 3185 cm–1 (figure 16A). Rubies from certain localities such as Mozambique and some pink sapphires from Madagascar show similar features when heat treated at lower temperatures (Smith, 1995; Vertriest and Saeseaw, 2019). While low-temperature and high-temperature heat treatment have been common for ruby and sapphire for decades, a new type of treatment has emerged in the last few years to improve the blue color of sapphires heated in the presence of pressure (~1 kilobar) (PHT). FTIR has once again proven useful, as a broad ~3045 cm–1 peak often appears after this treatment (Thanong et al., 2016; Peretti et al., 2018) (figure 16B). It is important to note that the presence of these peaks in FTIR spectra is useful for heat treatment detection, but their absence is not conclusive in any way. Conversely, the presence of a feature at 3161 cm–1 is a reliable indicator that a sapphire has not been treated at high temperatures. This peak is particularly valuable in ruling out beryllium diffusion treatment. Another application of FTIR for heat treatment detection in corundum is the identification of mineral inclusions in ruby or sapphire that are unstable at treatment temperatures. Certain secondary minerals in the form of aluminum hydroxides are commonly found in some gem corundum. These minerals (diaspore, gibbsite, and boehmite) have distinctive IR absorptions between 2300 and 3800 cm–1 in FTIR spectra. When present, they conclusively indicate that the ruby or sapphire has not been heat treated (figure 16C).
Emerald. Emerald is the bluish green to green variety of the mineral beryl. Due to emerald’s appeal, scientists began devising ways to grow them in a laboratory as early as 1848 (Koivula and Keller, 1985). There are two main methods to produce laboratory-grown emerald: flux growth and hydrothermal growth. Both types can be difficult to distinguish from natural stones by the untrained eye, and FTIR is a useful tool for distinguishing natural from laboratory-grown emeralds. Flux emeralds are grown from a hot molten metal flux, and their FTIR spectra are easily distinguishable due to the absence of water-related absorptions (~3400–4000, 5000–5500, and 6700–7500 cm–1). Both natural and hydrothermal laboratory-grown emeralds are grown from hot circulating fluids, making their FTIR spectra similar. Fortunately, a series of IR peaks from 2400 to 3100 cm–1 attributed to chlorine occur in the most common lab-grown hydrothermal emeralds, making identification possible (Stockton, 1987) (figure 17). FTIR can also aid in identifying fracture-filling materials in emerald (Johnson et al., 1999).
Alexandrite. Alexandrite is a color-change variety of the mineral chrysoberyl. The most sought-after color change is from a greenish hue under daylight or fluorescent lighting to a reddish hue under incandescent light. This dramatic effect has inspired its growth in a laboratory. Alexandrite can be synthesized by three different methods: flux-melt, floating zone, and Czochralski crystal “pulling.” All three involve crystallization from a molten liquid and yield comparable FTIR spectra. Conversely, natural alexandrite is formed in a metamorphic geological environment where fluids are abundant. Water-related IR absorptions in the crystal structure distinguish natural alexandrite from its lab-grown counterparts (Stockton and Kane, 1988).
Jadeite. Not all gemstones are single crystals of minerals. The term “jade” refers to two varieties of massive crystal aggregates that are commonly green in color: nephrite (composed of the amphibole minerals actinolite and tremolite) and the more valued and sought-after variety, jadeite. Like many other gemstones, much of the mined jadeite is not of high quality, containing uneven or little color and brown-stained fractures. As a result, a common practice is to bleach away the brown color using acids and fill the void spaces created with polymer resins to stabilize the stones for cutting and polishing. In some cases, colored dyes are mixed with the polymer to add color throughout the stone. This treatment is known as polymer impregnation with or without dying. While sometimes difficult to see with the untrained eye, the added polymers are readily visible in FTIR spectra, allowing identification of the treatment (Fritsch et al., 1992) (figure 18, top).
Turquoise. Another polymer-impregnated gem material is turquoise, a popular opaque blue to green ornamental gemstone. Finding large, intact pieces of good color is rare because it is a soft, often powdery aggregate of tiny mineral crystals that falls apart easily. To improve its stability, lower-quality turquoise is bonded together at the granular level using polymer resins. Because turquoise is opaque, IR light cannot pass through gem-sized pieces. Industrious gemologists, however, developed a technique to scrape small amounts of powder from the stones and mix them with KBr to form a solid translucent film that can be analyzed using FTIR. In this manner, FTIR spectra clearly identify the presence of polymer resins, even when they are difficult to see using a microscope (Liu et al., 2021) (figure 18, bottom). More recently, ATR FTIR has proven effective in place of KBr for turquoise (Čejka et al., 2015). Turquoise is also often imitated by dyeing common and inexpensive minerals such as howlite or magnesite, and FTIR spectra clearly distinguish turquoise from these imitations.
Other Gemstones. The benefits of FTIR analysis extend to many other gemstones as well. Using the KBr method described above, amber from the Baltic region can be separated from amber from the Dominican Republic and other sources through observation of a unique broad IR absorption around 1250 cm–1 (Abduriyim et al., 2009; Khanjian et al., 2013) (figure 19). Rare red beryl can also be differentiated from imitations and other beryl varieties using FTIR. Manufactured glass, which is commonly used as a gemstone, can be distinguished from naturally occurring glasses such as moldavite and obsidian using FTIR analysis (figure 20). Finally, the most abundant mineral on earth, quartz, has many color varieties (amethyst, citrine, rose, smoky) and is one of the most commonly faceted gem materials. FTIR spectra are useful in distinguishing some natural quartz from its lab-grown equivalent (Balitsky et al., 2004; Karampelas et al., 2011). These examples illustrate the wide-ranging role of FTIR analysis in gemology.
Gemstone Identification Using Reflection IR. While most FTIR analysis of gems is performed by some form of absorption spectroscopy, it is important to mention that reflection IR, particularly specular reflection (in which the IR beam simply reflects off of the gem surface rather than sampling the interior), can also be a very useful gem identification tool (Hainschwang and Notari, 2008). These direct reflection IR spectra often show different patterns compared to absorption spectra of the same gem, and they tend to be very distinctive for most gemstones. With consistent sampling protocols and a carefully curated reference library of reflectance spectra of known gemstones, this technique can provide a quick and effective method to identify gem materials. IR absorption spectroscopy is more useful for treatment detection and separating natural from laboratory-grown gems, but reflection spectroscopy provides a quick and reasonably reliable method to identify the gem mineral being examined.
CONCLUSIONS
Infrared spectroscopy is an indispensable tool for the effective and efficient identification of natural and laboratory-grown gemstones as well as many treatments applied to enhance their color. The vibrational properties of bonds between atoms in a mineral in response to IR energy produce distinctive and consistent absorptions that are easily observed in FTIR spectra taken at room temperature. This analytical technique has been a mainstay in gem laboratories since the 1990s and continues to be a valuable gemological tool. From measuring nitrogen impurities that define diamond type to detecting heat treatment features in ruby and sapphire and polymer impregnation of turquoise and jadeite and beyond, FTIR is an essential resource for gem identification.