Composition and Spectral Characteristics of Porcelain-Treated Turquoise

ABSTRACT
To meet the ever-increasing demand for high-quality natural turquoise, a novel treatment technique utilizing an inorganic additive appeared in the Chinese market around 2019. Turquoise with this treatment bears a strong resemblance to high-quality natural turquoise, with a fine structure and high surface luster, and is known in the Chinese trade as “porcelain-treated” turquoise. This material was characterized by routine gemological methods, environmental scanning electron microscopy, and spectroscopic methods including infrared, ultraviolet/visible, energy-dispersive X-ray fluorescence, and laser Raman spectroscopy. The infrared and ultraviolet/visible reflectance spectra of porcelain-treated turquoise are difficult to distinguish from untreated turquoise. However, porcelain-treated turquoise can be effectively identified by its surface features in combination with low specific gravity, strong luster, high silica concentration (>8.29 wt.%), high atomic ratio of iron to aluminum (>0.22), and low atomic percentage of phosphorus (<37.5%).
Turquoise is hydrous copper aluminum phosphate with a general chemical formula of CuAl6(PO4)4(OH)8•4H2O. It is widely distributed across localities such as China, Egypt (Mansour, 2014), Chile (Evans and Southward, 1914), the United States (Harbottle and Weigand, 1992; Hedquist 2016), Iran (Beale, 1973; Ovissi et al., 2017), and Mexico (Zalinski, 1907; Weigand et al., 1977). The main Chinese sources are Hubei Province (Chen et al., 2012), Shanxi Province (Luo et al., 2017), the Xinjiang Uyghur Autonomous Region (Liu et al., 2018), Anhui Province (Chen and Qi, 2007), and Henan Province (Zhou and Jiang, 2005). Zhushan County in Hubei is the world’s largest commercial source, and material from there usually develops dark iron veins because of its association with phosphate, iron, and copper ores (Tu, 1997).
Turquoise with various colors, fine texture, and moderate hardness has generally been used as carving material. The earliest turquoise artifacts in China were found at the Jiahu site in Wuyang County, Henan Province, and were dated back to 7000–5800 BCE (Yang et al., 2017). Porcelain is a world-renowned Chinese invention that originated from pottery around the middle of the Shang Dynasty in the sixteenth century BCE (Song, 2008). The term “porcelain” is used in China to describe the finest luster of turquoise, and high-quality material with such luster is referred to as “natural porcelain” turquoise (box A). This term was adopted because of the resemblance to the soft, bright, and long-lasting luster of porcelain. The luster of turquoise depends on the structure (density) of the material and the quality of polish.
In the current Chinese market, natural turquoise is broadly divided into three categories according to density: porcelain, medium-quality, and porous (box A). Porcelain turquoise is sought after for its high luster, uniform color, compact texture, and high hardness. Sky blue porcelain turquoise is regarded as the finest quality. Porous turquoise of low hardness and very light color is regarded as the lowest in quality.
Turquoise is typically a polycrystalline aggregate. Its various colors are attributed to the differences in the type and content of its constituent elements (Luan et al., 2004). Water content and porosity can also affect the color (Foord and Taggart, 1998; Chen, 2009; Liu, 2019). As weathering increases, both crystalline and structural water in turquoise are lost, resulting in a reduction in the structural integrity and a lightening of its color (Chen, 2009). If the space between grain boundaries is small, turquoise usually has low porosity, high density, high hardness, bright color, and strong surface luster. Conversely, if the space between microcrystals is large, the porosity will be high with low hardness (Chen, 2009), which will result in light-colored turquoise with low surface luster. These pores can be filled, to a certain extent, by wax/resin/polymer injection or immersion treatment methods to enhance luster and color. Pore filling not only facilitates turquoise processing but also increases stability and durability (Koivula et al., 1992; McClure et al., 2010; Ou et al., 2016).
In recent decades, the supply of high-quality turquoise has steadily fallen in the Chinese market (Chen, 2009), causing higher prices. Most low- and medium-quality natural turquoise has a light or uneven color, low luster, porous texture, and a tendency to crack (Chen et al., 2010a). These problems make it difficult to meet the ever-increasing consumer demand. Therefore, imitations and “optimized” (treated) products have been on the rise in the world market. Some merchants use other natural gemstone materials to imitate the color of turquoise by dyeing (Xie et al., 2010; Zhu et al., 2016; Schwarzinger and Schwarzinger, 2017), and there are a few synthetic versions available (Choudhary, 2010; Pristacz et al., 2013). However, these are not accepted in the Chinese market.
To enhance the color, texture, and density of low- and medium-quality turquoise, the main method is to inject an organic binder under atmospheric or higher pressures (Koivula et al., 1992; McClure et al., 2010). This process maximizes yield while improving durability. This treatment can be detected by infrared absorption spectroscopy, in which absorption bands are produced by the typical νs(CH2) symmetrical stretching vibration, νas(CH2) asymmetric stretching vibration, and ν(C=C) stretching vibration of organic polymers (Xu and Di, 2018).
Surprisingly, a novel technique utilizing an inorganic additive was introduced to the Chinese market in 2019. This method uses an inorganic binder to effectively improve the luster and color of low- and medium-quality material so that it resembles high-quality turquoise (figure 1). The study of this “porcelain-treated” turquoise is relatively new, and there are few reports on the process itself. Only Deng et al. (2019) have studied a specific porcelain treatment and the silicate solution used, in which the molar ratio of the sodium silicate to potassium silicate was 3.5:1.0. Meanwhile, many merchants treat turquoise to raise its price but do not disclose the details of the process. At present, the identification of porcelain-treated turquoise is challenging, and some labs may mistakenly identify it as untreated. Our investigation found that more than 10% of the turquoise in the Chinese jewelry market is treated with a silicate inorganic binder. Most porcelain-treated turquoise is sold as untreated, which is detrimental to the market.
MATERIALS AND METHODS
Samples. A total of 14 untreated and 26 porcelain-treated samples were examined in this study (table 1). All the samples from Zhushan County were obtained through the long-term cooperation between our research group and miners from the local market. Samples from the Guangzhou jewelry market had no origin record. All 40 specimens were polished (figure 2). To study the internal color distribution and the compositional and structural changes of the porcelain-treated turquoise, five of the samples after treatment were cut in half and polished (figure 3). The specimens were divided into two groups: P (porcelain-treated) and N (natural-color untreated).
The porcelain-treated specimens in this study were sky blue, whitish blue, bluish green, yellowish green, greenish blue, brownish blue, and brownish green. Some displayed an uneven color distribution, and many (such as P4, P5, P8, and N1) had irregular white patches on the surface. All the porcelain-treated samples had a waxy luster (table 1, where H refers to half beads of porcelain-treated turquoise; see also figure 2).
Standard Gemological Testing. Specific gravity (SG) of all specimens was recorded using a hydrostatic balance. Microscopic observations were carried out with a Leica M205A stereo microscope. Both tests were conducted at the Gemmological Institute, China University of Geosciences in Wuhan (GIC).
Infrared Spectroscopy. Infrared spectroscopy analysis was carried out using a Bruker Vertex 80 Fourier-transform infrared spectrometer at GIC. A small amount of sample and KBr powder (weight ratio of 1:150) were mixed, ground, and pressed to make a KBr tablet for transmission spectroscopy. The following conditions were used to test the tablet: 220 V scanning voltage, 6 mm raster, 10 kHz scanning rate, 32 scans, 400–4000 cm−1 range, and 4 cm−1 resolution. Baseline corrections were made using Bruker’s OPUS spectroscopy software.
Ultraviolet/Visible (UV-Vis) Spectroscopy. A UV-Vis spectrophotometer (PerkinElmer Lambda 650 S) was used to test the samples’ color characteristics at GIC. The test conditions were as follows: surface reflection method, 1 nm resolution, 100 ms collection time, a deuterium lamp excitation source at a color temperature of 2850 K, and a wavelength range of 250–800 nm.
Energy-Dispersive X-Ray Fluorescence (EDXRF) Spectroscopy. Chemical composition was determined by energy-dispersive X-ray fluorescence using a ThermoFisher ARL Quant’x EDXRF analyzer equipped with a rhodium-palladium X-ray tube and a Peltier-cooled detector at GIC. The analyses were performed using a voltage of 0 to 50 kV, a current of 1.98 mA, and a spot size of 1.5 mm2. Testing was carried out based on the turquoise working curve established by Liu and Yang (2018). The details of the turquoise working procedures were established as follows: Liu and Yang (2018) conducted laser ablation–inductively coupled plasma–mass spectrometry (LA-ICP-MS) testing on a different set of 26 pieces of untreated turquoise from Zhushan, nine uniform color test positions were selected for each sample, and the average value was taken as the standard value of each sample. Twenty-one of 26 turquoise samples with a composition gradient were selected as reference standards, and the working curve of analytical elements was established. Ten elements (aluminum, silicon, phosphorus, potassium, calcium, copper, iron, vanadium, chromium, and zinc) were selected for examination.
Raman Spectroscopy. A Raman spectrometer (Bruker Senterra R200L) was used to explore the abnormally high SiO2 content in the porcelain-treated turquoise at GIC. The laser wavelength was 532 nm, the laser power was 20 mW, and the acquisition time was 30 s. The laser spot size was 50 μm, the resolution was 9–15 cm–1, and the test range was 34–1500 cm–1.
Environmental Scanning Electron Microscope (ESEM). A Quanta 200 environmental scanning electron microscope was used to observe the structural characteristics of the porcelain-treated specimens at the State Key Laboratory of Geological Process and Mineral Resources, China University of Geosciences in Wuhan (CUG). The test conditions were as follows: room temperature of 15° to 20°C, a relative humidity of <80%, an acceleration voltage of 20 kV, and a magnification of 7× to 106×. Secondary electron images were collected. Three porcelain-treated samples (P4, H2, and H3) and one untreated turquoise sample (N14) were selected to obtain cross sections of polished areas coated with 10–20 nm of gold using SEM.
RESULTS AND DISCUSSION
Gemological Properties. All the porcelain-treated and untreated turquoise samples were tested for their standard gemological properties.
Specific Gravity. Turquoise is a polycrystalline aggregate, and its surface pores easily absorb water. Therefore, specific gravity (table 1) should be recorded within 2 seconds after immersion (He et al., 2018). The SG of the untreated samples ranged from 2.35 to 2.69, with an average of 2.57. There was a limited number of untreated turquoise samples in the test set, and previous studies have shown that the SG of untreated turquoise with a poor texture is typically less than 2.50, while turquoise with a high-quality texture generally has an SG greater than 2.70 (Chen, 2009; Xu et al., 2021).
The SG of the porcelain-treated specimens ranged from 1.89 to 2.56, with an average of 2.26 (figure 4). The porcelain-treated whitish blue samples’ SG range was 1.89–2.38 (average of 2.05), while the untreated whitish blue samples’ range was 2.35–2.61 (average of 2.51). The porcelain-treated sky blue samples’ SG range was 2.33–2.63 (average of 2.44), while the untreated sky blue samples’ range was 2.62–2.68 (average of 2.65). Untreated sample data from Chen (2009) and Xu et al. (2021) are also in this range. As can be seen, the average SG of porcelain-treated turquoise (both whitish blue and sky blue) was lower than the average SG of untreated turquoise.
The luster and hardness of untreated turquoise are related to its density (Wang, 1986; Foord and Taggart, 1998). The denser the structure and the higher the hardness, the stronger the luster. Untreated porcelain turquoise shows a strong waxy to glassy luster after polishing, while porous material has an earthy to weak waxy luster. The porcelain-treated specimens in this study generally had a higher luster (waxy to weak porcelain). Their density was similar to that of low-quality turquoise typically chosen for treatment. Hence, the combined characteristics of low density and strong surface luster can be used to identify porcelain-treated material.
Morphological Characteristics. Most of the porcelain-treated specimens purchased at the Zhushan market were whitish blue. They had waxy luster and a uniform color distribution, such as sample P1 in figure 2. The porcelain-treated turquoise specimens purchased in the Guangzhou market had various colors, including sky blue, whitish blue, and yellowish green. Some of the samples from both markets had high color saturation and waxy luster, such as samples P2–P10 in table 1.
Microscopic examination of porcelain-treated specimens P1, P5, and P10 showed obvious granular transparent crystals and fibrous filaments at pore channels and near the depressions of iron lines (figure 5). Specimen P1 originally had a waxy luster, but one week in an ambient environment (humidity above 50%) dulled its luster (figure 6, left) and left a blue spot on the surface (figure 6, right). Specimen H4 had a nonuniform color distribution with deep color along the edge and a bleached appearance along the iron vein. We attributed this uneven distribution to immersion in the filling material during treatment (figure 7, left). A round, transparent blob of melt with a greasy luster appeared on the surface of specimen P10 (figure 7, right).
Magnification and SG testing revealed that the stability of porcelain-treated turquoise is related to the density of the rough material before enhancement. Higher density produces a more stable color and luster. Because turquoise with high density is less porous, the filling material does not easily come out of the pores and the treatment is relatively stable.
Chemical Composition Analysis. All the porcelain-treated samples (P1–P21 and H1–H5) and ten of the untreated specimens with good luster (N1–N5 and N7–N11) were measured for major elements with EDXRF. All the porcelain-treated turquoise and some untreated samples (N1–N4) were also analyzed by Raman spectroscopy. Two or three different locations with uniform color were selected for each specimen, and each test location was tested twice with the average value reported with detection limits in table 2. The main chemical compositions of the porcelain-treated and untreated turquoise were similar, composed mainly of CuO, Al2O3, and P2O5, with traces of FeOT (including Fe2O3 and FeO), ZnO, SiO2, K2O, and CaO. Turquoise’s ideal chemical formula is CuAl6(PO4)4(OH)8•4H2O, in which iron can replace some of the aluminum and zinc can replace copper (Zhang et al., 1984; Foord and Taggart, 1998; Chen, 2009; Liu, 2019). The ideal formula in weight percentage of oxides was 37.60% Al2O3, 34.90% P2O5, 9.78% CuO, and 17.72% H2O. The original EDXRF test results do not include water (the total oxide content is approximately 100%), so we accounted for water by multiplying by 0.8228 (100% minus the 17.72% attributable to water). The results of these calculations are presented in table 2 (note the approximately 82% total for all of the measured samples) and plotted in figure 8. Figure 8 plots the differences between the major elements of porcelain-treated and natural turquoise. Among them, sample N2 had a ZnO to CuO weight percentage ratio greater than 1.0, making it a copper-zinc turquoise (Foord and Taggart, 1998).
The silica concentrations of all of the porcelain-treated samples were above 8.29 wt.%, generally higher than that of untreated turquoise. The silica contents of all the untreated samples tested in this study were all lower than 8.29 wt.% (the silica content of most untreated turquoise is <3 wt.%) (figure 8). Occasionally, the silica content of untreated turquoise can be high due to the existence of associated minerals, such as quartz and some clay minerals. In those cases, the elevated silica content was caused by associated minerals, according to the Raman spectroscopy results (figure 9). It was inferred that the binder used in the porcelain treatment contained silicon.
The weight percentages of aluminum + iron, copper + zinc, and phosphorus were converted into atomic percentages and plotted as the vertices of a ternary diagram (figure 10). It can be seen that untreated and porcelain-treated turquoise occupy two different areas, with some overlap. The atomic percentage of phosphorus in untreated turquoise is generally greater than 37.5%, significantly higher on average than in porcelain-treated turquoise. Data of untreated turquoise from Xu et al. (2021) are in agreement with our findings (blue points in figure 10). Therefore, the relationships between aluminum + iron, copper + zinc, and phosphorus are a useful means of separation. In addition, two-dimensional separation plots were generated between zinc and copper and between iron and aluminum (figure 11, A and B). It is impossible to distinguish porcelain-treated turquoise through the content relationship of zinc and copper. Figure 11 (B and C) shows some separation between untreated and porcelain-treated turquoise. Because the Fe/Al ratio of porcelain-treated turquoise (>0.22) is higher than that of untreated turquoise, it can potentially be distinguished by its iron/aluminum ratio.
Among the tested samples, the silica content of untreated specimen N2 was as high as 8 wt.%. Microscopic observation found a light-colored mineral with a transparent glassy luster, which was identified as quartz by Raman analysis (figure 9). The Raman shifts of 1091 and 816 cm–1 were caused by asymmetric and symmetrical stretching vibrations of Si-O-Si, and the peak values were low. The bending vibration Raman shift of Si-O-Si was 474 cm–1, and its peak was the highest. All the characteristic scattering peaks were sharp, and the full width at half maximum was small, indicating high crystallinity (Krishnan, 1945; Krishnamurti, 1958). Comparing the Raman spectra of untreated and porcelain-treated turquoise, there is little difference in spectral peaks from previous studies (Čejka et al., 2015; Štubňa and Andrášiová, 2021) (figure 12). In the 2800–3000 cm–1 range, some porcelain-treated material exhibited a weak peak, while untreated turquoise did not. The peak at the position of 2800–3000 cm–1 may be attributed to the asymmetrical stretching vibration and stretching vibration of CH2 (Chen et al., 2010b). This peak often occurs in turquoise treated with an organic binder. This peak from porcelain-treated turquoise at the position of 2800–3000 cm–1 (figure 12) is weak due to low spectral resolution, so the material cannot be identified as porcelain-treated from this peak. Since the EDXRF test found a high concentration of silicon in the porcelain-treated turquoise, it was inferred that the fillings are inorganic (containing silicate).
To explore whether the high silica content is caused by the porcelain treatment, three test positions of equal distance from the edge to the center of the split samples in group P (figure 3) were measured with EDXRF. Each different test location was tested twice and averaged. The silica content near the edge was higher than that at the center, indicating that the high silica content of the porcelain-treated turquoise was due to the enhancement process (figure 13) and the observed variations from sample to sample were caused by the silicon-containing filling.
Infrared Spectroscopy Features. All the porcelain-treated and untreated turquoise specimens were measured with infrared spectroscopy. The obtained infrared absorption spectra were divided into two regions: 4000–1300 cm–1 (region I) and 1300–400 cm–1 (region II). In the ideal turquoise structure, group theory analysis shows that there are two PO43– units in nonequivalent positions, two H2O units in nonequivalent positions, and four OH units in nonequivalent positions. Different units have different vibration characteristics. The vibration modes and frequencies of OH, H2O, and PO43– units determine the infrared absorption spectra of turquoise (Lind et al., 1983; Fritsch and Stockton, 1987; Chen, 2009; Čejka et al., 2015).
Untreated Turquoise. The absorption characteristics of untreated turquoise in region I were consistent with previous research (Chen et al., 2007). The absorption near 3500–3400 cm–1 corresponds to the ν(OH) stretching vibration, the absorption near 3300–3000 cm–1 is a δ(H2O) stretching vibration, and the peak near 1640 cm–1 is the δ(H2O) bending vibration (figure 14, left).
Untreated turquoise showed four vibration mode frequencies of PO43– in region II. The 1200–1000 cm–1 asymmetric stretching vibration absorption peak was ν3(PO43–), the four-fold set of peaks with strong absorbance. There was a ν1(PO43–) symmetrical stretching vibration frequency near 900 cm–1, with weak absorption. A δ(OH) out-of-plane bending vibration frequency was located near 840 and 786 cm–1. The bending vibration frequency of ν4(PO43–) was near 646, 595, 549, and 476 cm–1, and a symmetrical bending vibration frequency of ν2(PO43–) was near 470–410 cm–1 with weak absorption. Its main peak was near 1100 cm–1 (figure 14, left).
Porcelain-Treated Turquoise. The porcelain-treated turquoise in region I showed an absorption peak caused by the stretching vibration of ν(OH) near 3511 and 3465 cm–1, an absorption peak caused by the stretching vibration of ν(H2O) near 3300 and 3087 cm–1, and an absorption peak caused by the bending vibration of δ(H2O) near 1640 cm–1 (figure 14, right). Those absorption peaks were basically the same as those of untreated turquoise (Farmer, 1974).
The infrared absorption spectroscopy characteristics of porcelain-treated turquoise in region II showed that 1160, 1108, 1060, and 1020 cm–1 were the asymmetric stretching vibration absorption peaks of ν3(PO43–) (figure 14, right). The symmetrical stretching vibration frequency of ν1(PO43–) was near 900 cm–1, the out-of-plane bending vibration frequency of δ(OH) was located at 840 and 786 cm–1, and the bending vibration frequency of ν4(PO43–) was near 649, 609, 580, and 476 cm–1. The symmetrical bending vibration frequency of ν2(PO43–) was located near 470–410 cm–1; its absorption was weak, and some samples did not show this peak. The main peak of most samples was 1108 cm–1, consistent with that of the untreated specimens.
According to Farmer (1974), the absorption peak caused by the ν(Si-O-Si) stretching vibration was near 1100–1000 cm–1 with strong absorption. The ν(Si-O-Si) asymmetric stretching vibration frequency was near 1089 cm–1 with strong absorption. The ν(Si-O-Si) symmetric telescopic vibration frequency splits at 800 and 781 cm–1, and the asymmetric and symmetrical variable angle vibrations were located at 696 and 464 cm–1, respectively. The asymmetric stretching vibration of phosphate ν3(PO43–) was located at the strong absorption of 1100–1050 cm–1, and the symmetrical stretching vibration of ν1(PO43–) was located at 970 cm–1, which is very weak. A weak bending vibration of ν4(PO43–) was located at the absorption wavelength of 630–540 cm–1, and a weak symmetrical bending vibration of ν2(PO43–) was located at the absorption wavelength of 470–410 cm–1 (Farmer, 1974).
The absorption peak of the ν(Si-O-Si) asymmetric stretching vibration was similar to that of the ν3(PO43–) asymmetric stretching vibration, the absorption peak of the ν(Si-O-Si) symmetrical stretching vibration was similar to that of the δ(OH) out-of-plane bending vibration, and the ν(Si-O-Si) symmetrical stretching vibration absorption peak was close to that of the ν4(PO43–) bending vibration absorption peak. It was difficult to distinguish the presence of Si-O-Si without careful comparison.
We detected no peaks of organic matter in the infrared spectrum, and the infrared absorption peak of Si-O was very similar to that of PO43–.
UV-Vis Reflectance Spectrum Features. All the porcelain-treated and untreated specimens were measured with UV-Vis spectroscopy. In turquoise, copper is in a distorted octahedral coordination with six oxygens in an edge-sharing arrangement with pairs of alumina octahedra, and iron presumably replaces aluminum (Zhang et al., 1984; Foord and Taggart, 1998; Chen, 2009; Liu, 2019). The broad and strong absorption band near 620–750 nm is produced by the d-d electron transition of Cu2+. The d-d electron transition of Fe3+ produces an absorption band near the violet region at 425 nm, while the weak absorption band in the ultraviolet region at 370 nm is caused by the Fe3+ electron transition and charge transferring from O2− to Fe3+ (Foord and Taggart, 1998; Chen, 2009). The UV-Vis reflectance spectra of porcelain-treated turquoise (figure 15) show a broad absorption band in the yellow to red region (620–750 nm) and two absorption peaks in the purple region (~422 and 429 nm), which are almost identical to the UV-Vis absorption spectra of untreated turquoise. N5 and P10 are greener than N1 and P2 and have more iron, so their absorptions are stronger at 422 and 429 nm.
Basis for Identifying Porcelain-Treated Turquoise Using Nondestructive Methods. Both untreated and porcelain-treated turquoise can be identified through nondestructive testing, focusing on the appearance and composition characteristics of porcelain-treated turquoise (see the flowchart in figure 16):
- the combination of strong surface luster and low density
- fibrous filaments and potentially an area with greasy luster on the surface
- high atomic ratio of iron to aluminum (>0.22) and low atomic percentage of phosphorus (<37.5%)
- high silica content (>8.29 wt.%) in at least five test sites.
The silicon content at multiple test locations (without evidence of minerals that would account for it) is the decisive basis for identification of porcelain treatment, while the surface features and atomic content are the auxiliary basis. In the identification process, however, it is still necessary to comprehensively consider all four factors.
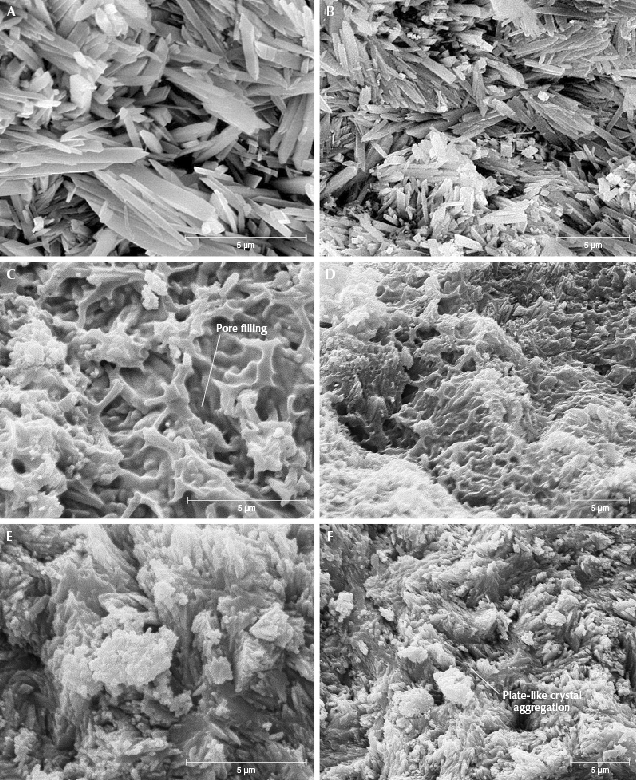
ESEM. Three porcelain-treated specimens with different densities (P4, H2, and H3) and one untreated specimen (N14) were examined with ESEM. Turquoise is triclinic and has a mostly cryptocrystalline structure with very large pores (Cid-Dresdner, 1965; Foord and Taggart, 1998; Chen, 2009; Abdu et al., 2011). Fine crystals can be observed under an electron microscope, mostly in the form of thin columns, plates, and scales. Figure 17 (A and B) shows the structure of untreated turquoise under 10,000× and 6,000× magnification, respectively. In specimens with different densities, there are also differences in the arrangements of microcrystals; turquoise with high density has a compact arrangement and small pores, whereas turquoise with low density has a loose, disorderly arrangement and large pores (Cid-Dresdner, 1965).
Three porcelain-treated specimens (P4, H2, and H3) were selected for SEM examination, and their structural characteristics were compared with those of untreated turquoise. For observation of each split specimen, three test positions were selected from edge to center. SEM images of porcelain-treated turquoise near the edge of the test sites are shown in figure 17 (C and D). Microcrystals within porcelain-treated turquoise lack the typical columnar and plate-like crystals of untreated turquoise aggregates. These microcrystals within porcelain-treated turquoise are approximately lath-shaped with a disorderly distribution, and they have rounded microcrystalline edges and blurred boundaries. Also, gel-like colloids (e.g., figure 17C) distributed between the pores of microcrystals and the crystal surface can be seen (the pores between crystals are no longer visible). As shown in figure 17 (E and F), the test location near the center was less affected by the porcelain treatment and the crystals are columnar and plate-shaped, with observable edges and pores.
The surface characteristics produced by porcelain treatment are obvious, while the effect within the stone is less evident, similar to the EDXRF results. SEM imaging shows that the morphology near the center of porcelain-treated turquoise is similar to that of untreated turquoise, with complete crystal shapes, obvious pores, and a disorderly arrangement of microcrystals. SEM is a useful technique to distinguish untreated from porcelain-treated turquoise through identification of microcrystal morphologies and colloid distributions. However, it is not suitable for routine gem identification because of its destructiveness.
CONCLUSIONS
Porcelain-treated turquoise can be reliably separated from natural turquoise if a certain combination of characteristics are present: low specific gravity, strong surface luster, surface features (including fibrous filaments, greasy melted areas, and color distribution), high silica content (>8.29 wt.%), a high atomic ratio of iron to aluminum (>0.22), a low atomic percentage of phosphorus (<37.5%), and evidence of colloid-filled pores with SEM examination. High silica content is the most diagnostic feature of porcelain treatment, while low SG combined with strong luster and high atomic ratio of iron to aluminum are auxiliary identification criteria. However, it is necessary to comprehensively evaluate all four factors to make a final determination. The infrared and UV-Vis absorption spectra characteristics are basically the same for untreated and porcelain-treated turquoise and therefore not useful for separation. SEM observation of surface micromorphology provides distinct evidence of porcelain treatment but requires coating with carbon or gold, making it unsuitable for routine identification.