Gems Formed in Magmatic Rocks
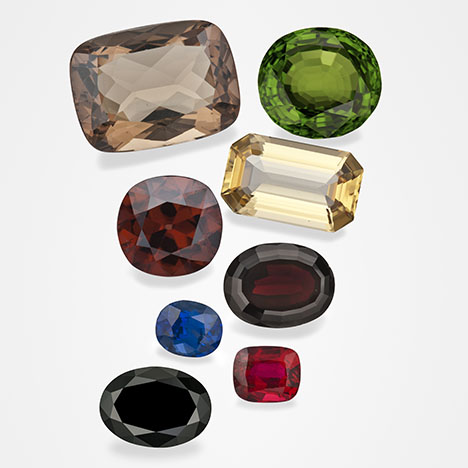
The rigid outer layers of the earth (the lithosphere) are composed of minerals and rocks. Minerals are natural chemical compounds that form in certain geological environments and sets of physical and chemical conditions. In the lithosphere, the main mechanisms for mineral formation are cooling and solidification from igneous magmas, crystallization from high-temperature hydrothermal solutions or vapors, crystallization from low-temperature aqueous solutions, and solid-state mineral recrystallization during metamorphism. Gem minerals can be formed by any of these mechanisms.
Minerals have specific chemical compositions and arrangements of constituent atoms (i.e., crystal structure). Approximately 5,800 mineral species are currently recognized by the International Mineralogical Association (IMA), most of them rare and narrowly distributed. Despite the variety of mineral species, almost 97% of the earth’s crust is made up of a few elements: oxygen, silicon, aluminum, iron, calcium, sodium, potassium, and magnesium. Hence, the crust is dominated by a small handful of minerals containing these elements (feldspars, quartzes, pyroxenes, amphiboles, and micas). While these minerals are occasionally found as gem-quality pieces, most natural gems are rarer mineral phases that fortunately occur in specific deposits as crystals of a size and quality that can be faceted or polished.
This edition of Colored Stones Unearthed will focus on gem minerals that form in magmatic environments (figure 1). Other environments of gem formation will be discussed in future columns.
MINERAL FORMATION IN MAGMAS
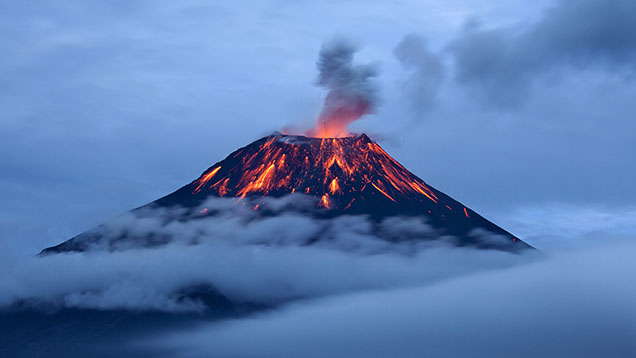
Magmatic minerals form during the cooling and crystallization of magmas (molten rock) in certain portions of the earth’s lithosphere and at the surface (figure 2). These magmas vary in chemical composition, but for most the dominant chemical component is silica (SiO2). The amount of SiO2 in a magma dictates many of the properties and eruptive behaviors of volcanic events, and silica concentration can tell researchers a great deal about the geological history of a magma. Basalts, for instance, are silica-poor magmas (typically ~50 wt.%), while rhyolites have high silica contents (typically >70 wt.%). Magmas can form when temperatures rise in the earth and melting of preexisting rocks occurs in the lower crust or upper mantle. Decreases in pressure or the introduction of water or other volatile components can also result in the production of magma deep within the earth. Once formed, magmas can either travel through the crust via fissures, form large magma-filled chambers in other rocks, or be erupted at the earth’s surface through volcanic vents (figure 3).
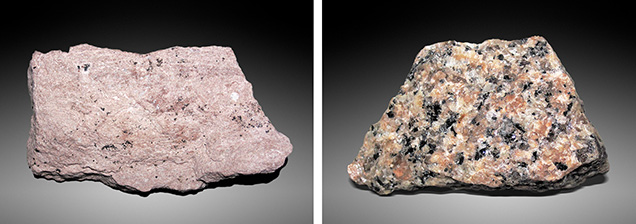
Whatever their origin, the cooling of magmas results in the crystallization of igneous rocks, which are classified according to their texture and mineral composition (table 1). As the magma’s temperature decreases and it begins to solidify, mineral formation can occur below the surface (to form intrusive or plutonic or phaneritic rocks with coarser grain size due to slower gradual magma cooling). Alternatively, magma that is erupted at the surface will solidify quickly, often leading to small grain sizes of the constituent minerals (to form extrusive or aphanitic rocks) (figure 4).
VOLCANIC ERUPTIONS
A volcano forms above an underground magma chamber and is created where the magma is vented or erupted at the earth’s surface. Volcanoes occur at specific locations of the crust that are associated with the creation, lateral movement, and then subduction of crustal plates. Most volcanoes are located along boundaries of crustal plates, either at divergent plate boundaries (mid-ocean ridges or continental rift zones where crustal plates separate) or convergent plate boundaries (subduction zones where plates collide). Some volcanoes also occur within crustal plates where they form above “hot spots,” anomalously hot areas of the upper mantle. Below Hawaii, for example, successive islands were created as the crustal plate gradually moved over a thermal feature where magmas were generated (figure 5).
Depending on their chemical composition, silica-poor magmas like basalts are very fluid when erupted (and nonexplosive), and they flow down the gentle slopes of volcanoes as molten lava (a shield volcano such as Mauna Kea in Hawaii). Other, more silica-rich magmas like rhyolites are much more viscous and explosive. When erupted, they form higher steep-sided volcanoes that often produce violent ash cloud explosions or pyroclastic debris flows, depositing the particle material along the margins of the volcano or over the surrounding countryside (e.g., a stratovolcano such as Mount Vesuvius in Italy).
Magmas may solidify underground to form various types of intrusive igneous rock bodies. Depending on size and shape, geologists use terms to describe these rock bodies (all of which may be later exposed at the earth’s surface by geological processes such as folding, faulting, crustal uplift, and weathering; see again figure 3):
- Dike: a narrow rock body that cuts across other rocks
- Sill: a narrow rock body that runs parallel to sedimentary layers
- Diapir: a domed body of igneous rock that has been forced upward into brittle overlying rocks
- Pluton (or batholith): a large mass of igneous rock
- Laccolith (or lopolith): a body of igneous rock that bulges upward or downward
MAGMA CRYSTALLIZATION
Minerals in the crust crystallize from magmas over a range of temperatures (~1300° to ~750°C). Most magmas are silica-based compositions and classified according to their silica content, from silica-poor magmas like basalts (~50 wt.% SiO2) to intermediate magmas like andesites (~60 wt.% SiO2) to silica-rich magmas like rhyolites (>70 wt.% SiO2). Silicate magmas are dominated by oxygen and silicon, and they contain lesser amounts of aluminum, calcium, magnesium, iron, sodium, and potassium as well as rarer elements. Non-silica-based magmas such as carbonatites are much less common and can form only under specific geological conditions.
The silica tetrahedron (SiO4) is the basic building block of all silicate minerals. It consists of a central silicon atom bonded to four oxygen atoms that form a tetrahedral arrangement. Silica tetrahedra can also bond to one another by sharing one or more of their oxygen atoms to form more complex tetrahedral arrangements (such as pairs, single or double chains, rings, sheets, and frameworks of bonded tetrahedra; see figure 6). These tetrahedra are further connected by atoms of other elements to form the crystal structures of various silicate minerals such as olivine, epidote, pyroxenes, amphiboles, beryl, mica, and feldspars, to name a few.
Several geologic factors influence the evolution of magma composition and crystallization. These include the initial composition of the magma source, the presence of volatiles such as water or elements like fluorine in the magma, partial melting of rocks at the source, interaction with melted or unmelted (restite) minerals, interaction with or assimilation of wall rocks, mixing of magmas, separation of magmas into immiscible melts, loss of volatile phases (degassing), fractional crystallization of minerals, and accumulation of early-formed minerals. As a result of these factors, the overall composition of the magma, and the minerals that crystallize from it, changes over time. Common silicate mineral formation takes place from the magma at progressively cooler temperatures in a specific sequence. This sequence is schematically described by Bowen’s reaction series (figure 7). This reaction series illustrates the differences between minerals that typically crystallize from magmas at high temperatures (e.g., olivine, pyroxenes, amphiboles, micas, and calcium-rich feldspar) and those that typically crystallize at lower temperatures (quartz, micas, and potassium-rich feldspar). If early-formed minerals are separated from a magma, the removal of those components changes the composition of the remaining magma, generally leading to more silica-rich compositions (figure 8). Bowen’s reaction series can also be considered from the bottom up in that the lower-temperature minerals at the bottom of the diagram are also the first to break down during partial melting. The presence of silica-rich minerals like quartz, micas, and potassium-rich feldspar at the bottom of the diagram demonstrates that the early-formed magmas during partial melting tend to be silica-rich. Removal of such partial melts can produce rhyolitic magmas.
Magmatic rock environments are also important sources for ore deposits—economic and minable concentrations of gold, silver, copper, nickel, platinum, chromium, vanadium, cobalt, and other elements that are not found in large amounts in most silicate minerals.
Hydrothermal and pegmatitic deposits of gems, although related to magmatic activity, will be discussed separately in future editions of this column because of their importance for certain gem deposits.
GEM MINERAL FORMATION FROM MAGMAS
Magmatic gems form either by crystallization of the magma at depth or at the earth’s surface, and occasionally in rock cavities from a gas phase (a vapor) released during magma cooling. Magma eruptions can also bring up mineral crystals or fragments of deeply formed crystals or rocks (including some gem minerals) derived from other sources—geologists refer to them as xenocrysts or xenoliths.
When exposed at the surface, igneous rocks often weather and decompose to form smaller particles. Resistant minerals (such as diamond or corundum) can remain in the sedimentary environment and become concentrated in secondary alluvial deposits by the movement of water. Such deposits are sources for several important gem minerals. Over time, long-distance water transport of gem crystals also can winnow out fractured crystals: Those that survive the journey can be waterworn but of higher quality.
Although gem mineral formation from magmas is the subject of this column, some of these same minerals can also form by other geological processes such as metamorphism.
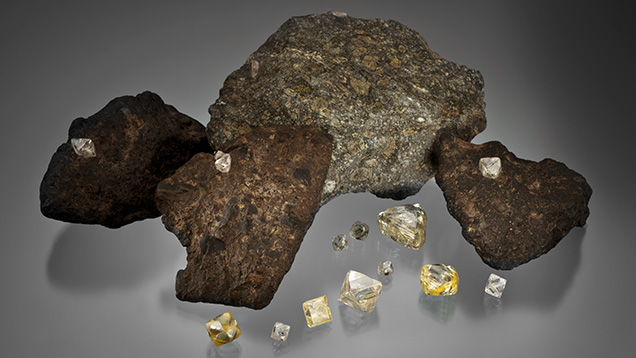
Diamond. Most gem diamonds formed between 1.0 and 3.5 billion years ago and at depths between 150 and 250 km in the lower crust and upper mantle (and in some cases in the lower mantle as deep as 800 km; see Smith et al., 2017). It is now understood that their formation took place from carbon-containing fluids, based on studies of tiny inclusions of minerals and fluids found within diamonds, although the details of this growth process are still the subject of geological study. Once formed, the diamonds were carried to the earth’s surface by eruptions of kimberlitic or lamproitic magmas (figure 9). Deeply formed diamonds were slowly transported upward by convection in the mantle to a depth where they could be caught up in erupting igneous magmas. Diamond formation was described in more detail by Shirey and Shigley (2013) and by Smit and Shirey (2018, 2019). At the surface, diamonds are recovered by mining of primary kimberlite ore and from secondary alluvial deposits. Harris and Staebler (2017) and Smit et al. (2022) provided recent reviews of diamond geology, genesis, and occurrence.
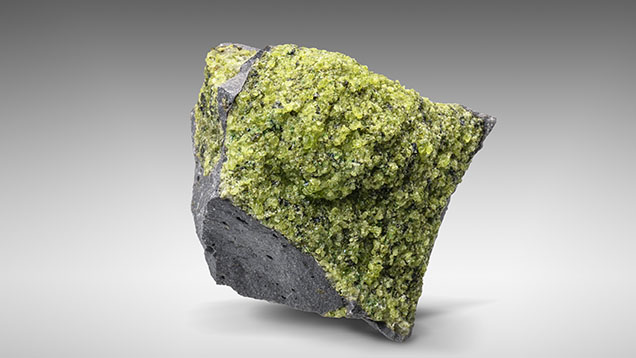
Olivine (Peridot). Olivine is a magnesium-iron silicate mineral with the formula (Mg,Fe)2SiO4 and a crystal structure consisting of isolated silicate tetrahedra joined by metal cations. It is one of the first minerals to crystallize from silicate magmas, and it occurs in mafic (low SiO2, high MgO and FeO) and ultramafic (very low SiO2 at <45 wt.% and high MgO and FeO) igneous rocks that are low in silica such as gabbro and basalt. Magnesium-rich olivines (forsterite) are more common than iron-rich olivines (fayalites) and constitute most gem peridot. Olivines are primary components of the earth’s upper mantle (see again figure 8). Much of the peridot on the gem market comes from alkali basalt eruptions that originated in the mantle and carried up large chunks of mantle rocks (including olivine and pyroxene crystals) as xenoliths (Koivula, 1981). These constitute the major deposits in the U.S. state of Arizona (figure 10) and Jilin, China.
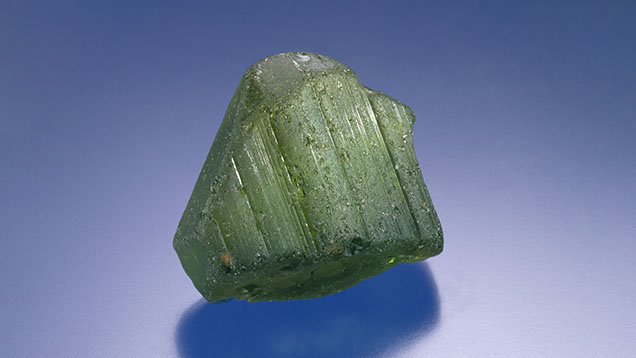
Other peridot deposits form as a result of hydrothermal fluid circulation through very magnesium- and iron-rich mantle rocks called peridotites, which were brought up to the earth’s surface during continental collisional events. These are represented by the deposits in Myanmar, Pakistan, and the classical historical source of gem peridot on Zabargad (Saint John’s) Island in the Red Sea of Egypt (figure 11). However the olivine reaches the surface, it quickly weathers and decomposes to form clays.
Rare iron-nickel meteorites that contain crystals of olivine are known as pallasites (Sinkankas et al., 1992; Shen et al., 2011). Several recent publications have discussed olivine formation in magmatic igneous rocks as well as gem peridot (Revheim, 2015; Wilson, 2020; Wallace et al., 2021; Seneewong-Na-Ayutthaya et al., 2021).
Quartz. Quartz (SiO2) and its color varieties (mainly amethyst, citrine, rose quartz, and smoky quartz) have been gem materials since ancient times as crystals, crystal fragments, and cryptocrystalline aggregates (such as chalcedony and agate). It is a principal constituent of granites and other silica-rich igneous rocks where it has crystallized from molten magmas. Amethyst-filled cavities and geodes in basaltic lava flows are an important source of gem crystals. Quartz is resistant to weathering and is commonly found as fragments, sometimes waterworn, in alluvial sediments and soils.
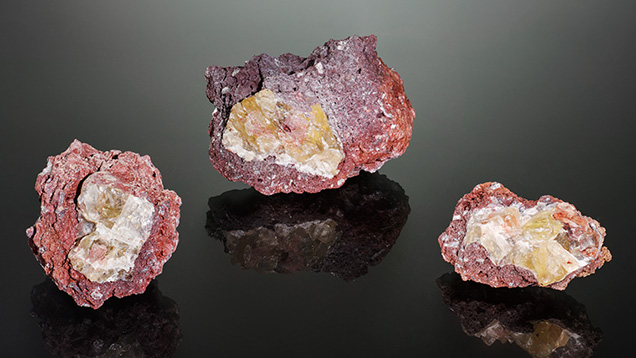
Feldspar. Feldspars are an important group of potassium-sodium (alkali feldspars: [K,Na]AlSi3O8) or sodium-calcium minerals (plagioclase feldspars: NaAlSi3O8–CaAl2Si2O8) whose structures are based on a three-dimensional network of silica or alumina tetrahedra. They exhibit extensive chemical solid solutions within the group. Feldspars crystallize from a variety of magma compositions, beginning with more calcium-rich phases to more sodium-rich phases with decreasing magma temperatures (figure 8). Potassium-rich feldspars are among the last minerals to form from a magma. Feldspars are important constituents of both intrusive (granites or diorites) and extrusive (rhyolites or andesites) igneous rocks. As a group, they compose about 60% of the earth’s crust. Feldspars weather to form clay minerals and are not usually found in alluvial deposits, as they are not resistant enough. They are commonly opaque but sometimes occur as transparent crystals suitable for faceting (orthoclase, microcline, and oligoclase; see figure 12) or as crystals that exhibit optical phenomena such as aventurescence (sunstone, or rainbow lattice sunstone; Johnston et al., 1991; Pay et al., 2013; Jin et al., 2022), adularescence (moonstone), or iridescence/labradorescence (labradorite) that are cut as faceted stones or cabochons.
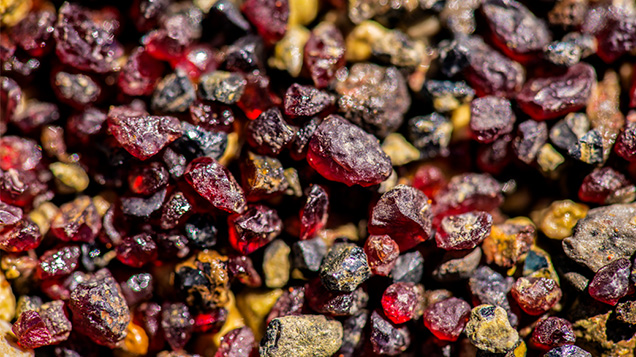
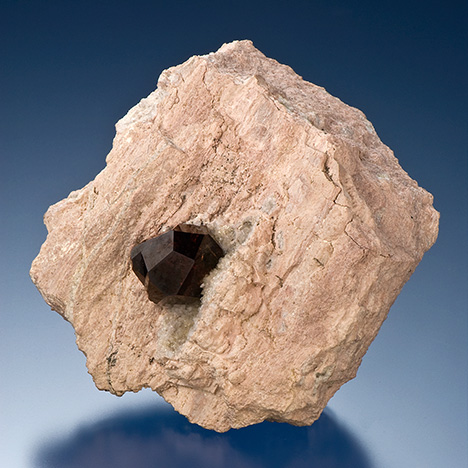
Garnet. Garnets are an important group of approximately 20 minerals represented by several valuable gemstones such as demantoid, hessonite, rhodolite, and tsavorite. They exhibit a wide variation in chemical composition—the common species are often referred to as “pyralspite” garnets [(Mg,Fe,Mn)3Al2(SiO4)3] and “ugrandite” garnets [Ca3(Cr,Al,Fe)2(SiO4)3]. Some garnets can display a color change under different lighting conditions, while others exhibit optical phenomena such as asterism because of symmetrically oriented acicular inclusions. Some garnet species are common minerals, while others occur less frequently. Like olivine, the crystal structure of garnet consists of isolated silica tetrahedra linked by a variety of metal cations in several structural sites. They occur widely in metamorphic rocks, and to a lesser extent as an accessory phase in igneous rocks such as granites, granitic pegmatites, gabbros, and basalts. Pyrope is an important indicator mineral used in the search for diamond-bearing kimberlites (figure 13). The mineral also forms in the crust or upper mantle and is brought up to the surface by ascending magmas or by geologic uplift of deep rocks. While most gem-quality garnet on the market would be considered metamorphic or hydrothermal in origin, on occasion they are found in cavities of erupted igneous rocks like rhyolites, where they were formed by gases released by the cooling magmas (figure 14). Garnets are resistant to abrasion and chemical weathering, so they can remain in sediments and become concentrated in alluvial deposits. A review of garnets and their geological occurrences was provided by Gilg et al. (2008).
Spinel. The magnesium-aluminum oxide spinel (MgAl2O4) is one species in a group of minerals referred to by the same name that have a similar crystal structure but varied chemical composition. Depending on the minor elements present, it occurs in a variety of colors, with the red variety long being recognized as a gemstone that in earlier times was often referred to as “carbuncle” or “Balas ruby” (“Badakhshan ruby” from Tajikistan) in confusion with ruby. Spinel is a common mineral in metamorphic rocks, but it can also form as an accessory mineral in mafic igneous rocks that are poor in silica and alkali elements. The conditions of formation of a silica-free oxide mineral such as spinel from a silica-containing mafic igneous magma are still a matter of debate among geologists. Spinel is an important constituent of peridotite in the upper mantle and in kimberlites. Because of its hardness, lack of cleavage, high specific gravity, and resistance to weathering, spinel is found concentrated in secondary alluvial sediment deposits.
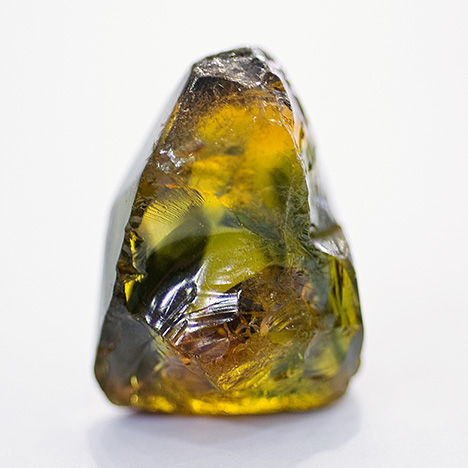
Corundum. Ruby and the many colors of sapphire of the mineral corundum (Al2O3) are among the most important and valuable gemstones because of their occurrence as colored transparent crystals suitable for faceting or polishing. Corundum is colorless when pure, but the potential presence of minor elements (namely chromium, titanium, and iron) can act as coloring agents (Dubinsky et al., 2020). The structure consists of a hexagonal close-packed arrangement of oxygen atoms with aluminum and/or other elements occurring in structural sites. The gem material may display asterism and color-change behaviors. Corundum is found in metamorphic rocks as well as low-silica syenites, pegmatites, and other less common igneous rocks (Simonet et al., 2008; Palke et al., 2019a,b). The mineral also occurs as xenocrysts in igneous alkali basalts; however, rapid weathering of basalt at the earth’s surface means that most ruby and sapphire of igneous origin are encountered as loose crystals or fragments in alluvial sediments (figure 15). As with spinel, the conditions of formation of a silica-free oxide mineral such as corundum from a silica-containing mafic igneous magma remain a matter of debate among geologists (Giuliani and Groat, 2019). Nonetheless, sapphire and ruby from the alkali basalt–related deposits have glassy silicate melt inclusions that represent some primordial magma from when the gem corundum was growing. This indicates that although the sapphire and ruby were xenocrysts in the alkali basalts that carried them to the surface, they must have a magmatic origin from deep within the earth. Because of its toughness, hardness, and resistance to weathering, and because the igneous host rocks decompose at the earth’s surface, corundum is often found as a detrital mineral in sediments and secondary alluvial deposits.
Zircon. Zirconium is an uncommon element in the earth’s crust, so zircon (ZrSiO4) only occurs as small accessory crystals or grains in a wide variety of rocks. Larger transparent crystals are less common, so gem zircon suitable for faceting is rare. The structure is composed of isolated silica tetrahedra bonded together by zirconium atoms. The mineral is normally reddish to yellowish brown, but other colors such as blue and green also occur as a result of trace elements in the crystal structure, radiation exposure, or natural or artificial heating. The mineral’s hardness and chemical resistance to weathering mean that it is frequently preserved in sediments and sedimentary rocks. Most of the gem-quality zircon on the market is related to alkali basalt deposits where the zircon are found as xenocrysts. This is similar to the deposits of magmatic gem corundum, and both are often found in the same deposit.
Because they often contain small amounts of radioactive uranium or thorium, zircon crystals provide an important way to date the geologic age of their host minerals or sediments, including some of the oldest minerals dated so far (4.4 billion years for zircon from one locality in Australia). The presence of radioactive minerals causes damage to the crystal structure, so these zircon are described as metamict with an opaque appearance and lower hardness, refractive index, and specific gravity.
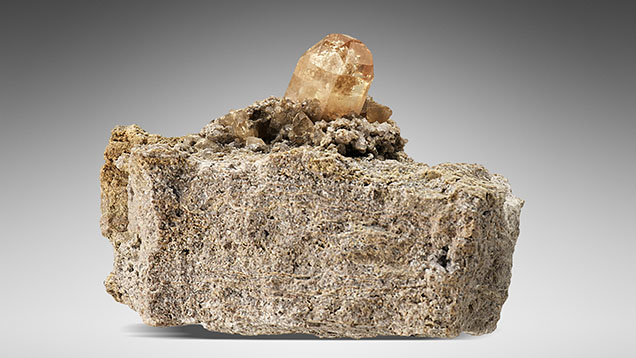
Topaz. Topaz, with the formula Al2SiO4(F,OH)2, is an aluminosilicate mineral that contains fluorine and/or hydroxyl (OH) ions. The requirement for fluorine limits the range of geological environments for topaz formation. This mineral occurs in open veins or voids forming at low to moderate temperatures in later-stage, silica-rich igneous rocks such as granites, pegmatites, and rhyolites in which a fluorine fluid or vapor appears to have been present (such as the historical topaz occurrence at Schneckenstein, Germany; Zeug et al., 2022). Certain rare granites are so enriched in fluorine that topaz is an essential mineral constituent of the rock. Topaz crystals that grow from late-stage magmatic vapors are also found in cavities in rhyolites (figure 16). Although it is not as hard as other gem minerals and has a tendency to cleave perpendicular to the long axis of the crystal, topaz is resistant enough to be preserved as waterworn crystals or fragments in alluvial sediments. Often colorless, the mineral can also be blue, yellow, brown, or reddish orange. The crystals are usually transparent and sometimes large. Staebler et al. (2011) reviewed the geological occurrence of this gem mineral.
INCLUSIONS IN MAGMATIC GEMS
Gems that crystallize from cooling magmas can exhibit micro-features visible with a gemological microscope. In addition to solid inclusions (such as oriented needles in a magmatic ruby or sapphire), probably the most distinctive micro-feature is a rounded or irregular melt inclusion, which represents a solidified bubble of the silicate magmatic melt present when the host gemstone was crystallizing. Upon cooling, the melt was quenched to a glass, which may contain a gas bubble and perhaps tiny crystals that formed from the glass. Figure 17 presents a compilation of melt inclusions that offer proof of a magmatic geological origin.
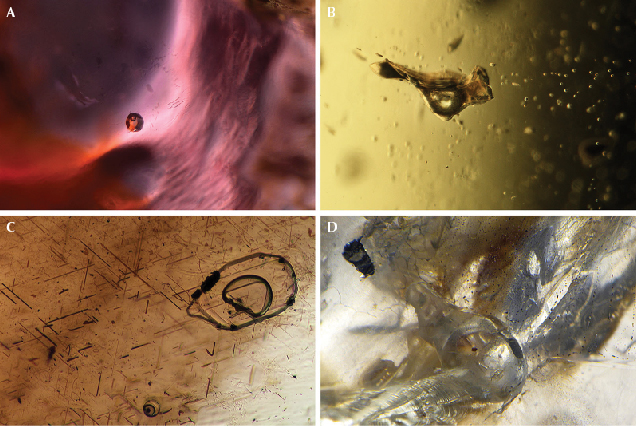
CONCLUSIONS
From partial melting deep within the earth to post-emplacement processes in volcanic eruptions, many of the world’s finest gemstones were derived from magmatic activity. The study of these gemstones can provide geological insights into the generation of magmas within the earth, their transport to the earth’s surface, and modes of volcanic processes after eruption. Magmatic gemstones offer many clues to the earth’s evolution and the geological processes that shaped our world. The next installment of Colored Stones Unearthed will focus on gemstones formed through metamorphic processes.