Kimberlites: Earth’s Diamond Delivery System
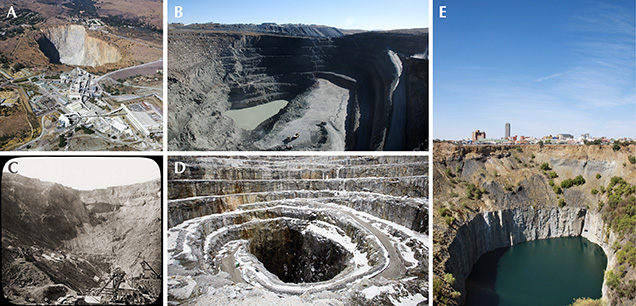
Diamonds are the most amazing of gems. Just as amazing, however, is how natural diamonds reach Earth’s surface. Diamonds are formed 150 to 700 km deep in Earth, and are then carried upward in a rare volcanic eruption of a kimberlite magma. Man has never witnessed such an event, and the eruption of this magma is thought to be the most rapid and violent type of volcanic eruption on Earth. Luckily, since diamond is the hardest mineral, it can usually survive such rough handling. This delivery system in the form of volcanic transport only adds to the mystique and value of natural diamond.
There are two main magma types that carry natural diamonds to the surface. These magmas crystallize on cooling into volcanic rocks known as kimberlite and lamproite (see box A). Kimberlite is by far the dominant type of eruption to bring diamonds to Earth’s surface (figure 1). Although diamond is only an accidental passenger and not actually created by the kimberlite, a basic understanding of kimberlites helps us understand the setting for most natural diamond formation in the mantle.
The Relationship Between Kimberlite and Diamond
Prior to the discovery of kimberlites, diamonds were all mined from secondary alluvial sources: river environments where diamonds had been eroded from their primary source. Historical diamonds from India were predominately recovered along the Krishna River in Madhya Pradesh. Today, secondary diamond mining still occurs in many areas of Sierra Leone, Brazil, Angola, Namibia, and even along the seafloor where rivers drain into the oceans.
The common occurrence of shale pieces in the first discovered kimberlite confused early geologists (see box B). Shale was a piece of the surrounding rock that had been picked up by the kimberlite as it traveled through the crust before eruption. Since shale is often very carbon rich, some geologists reasoned that diamonds might have formed by reaction between the magma and the shale (Lewis, 1887b). At the time, some 30 years before the discovery of radioactivity, there was no way to accurately determine the absolute age of a diamond (see Spring 2019 Diamonds from the Deep), the kimberlite, or the shale.
Box B: Discovery of Kimberlites as the Source Rock for Diamonds |
Between 1866 and 1869, the first South African diamonds were discovered along the Vaal and Orange River beds (known as “alluvial” diamonds). This was followed by the first discoveries of diamonds in their primary source rock at Jagersfontein, Koffiefontein, and the Kimberley area in 1870. Figure B-1 shows early mining operations at Kimberley. ![]() Figure B-1. Image of the haphazard mining operations at the Kimberley “Big Hole” before the consolidation of mining operations by Cecil Rhodes and Barney Barnato, and the founding of the De Beers Consolidated Mines in 1888. Each miner owned a small claim of land and sent diggings to the surface by winch and rope. They worked at different rates and left a highly irregular and dangerous surface. See also figure 1C.
Ernest Cohen first recognized this new source rock as igneous (Janse, 1985), and Henry Lewis (1887a) proposed to call the rock “kimberlite.” It was named after the town of Kimberley, which in turn was named after Lord Kimberley, the British Secretary of State (Field et al., 2008, and references therein). The observations of Lewis (1887b), extracted below, provide an interesting glimpse into the dawning understanding of the geologic conditions of diamond occurrences more than 130 years ago: In 1870, at which time some ten thousand persons had gathered along the banks of the Vaal, the news came of the discovery of diamonds at a point some fifteen miles away from the river, where the town of Kimberley now stands. These were the so-called “dry diggings,” at first thought to be alluvial deposits, but now proved to be volcanic pipes of a highly interesting character. Four of these pipes or necks, all rich in diamonds, and of similar geological structure, were found close together. They have been proved to go down vertically to an unknown depth, penetrating the surrounding strata. The diamond-bearing material at first excavated was a crumbling yellowish earth, which at a depth of about 50 feet became harder and darker, finally acquiring a slaty blue or dark green colour and a greasy feel, resembling certain varieties of serpentine. This is the well-known “blue ground” of the diamond miners. It is exposed to the sun for a short time, when it readily disintegrates, and is then washed for its diamonds. This “blue ground” has now been penetrated to a depth of 600 feet, and is found to become harder and more rock-like as the depth increases. The diamond-bearing portions often contain so many inclusions of shale as to resemble a breccia, and thus the lava passes by degrees into tuff or volcanic ash, which is also rich in diamonds, and is more readily decomposable than the denser lava. It seems evident that the diamond-bearing pipes are true volcanic necks, composed of a very basic lava associated with a volcanic breccia and with tuff, and that the diamonds are secondary minerals produced by the reaction of this lava, with heat and pressure, on the carbonaceous shales in contact with and enveloped by it. |
It took experiments and geochemical analysis to show that diamonds do not form as a result of kimberlite reaction with shale. But it would take more than 100 years after the discovery of kimberlite to prove that diamonds do not crystallize out of the kimberlite magma.
The first step in our knowledge about the relationship between diamonds and kimberlites comes from early work on how diamond crystallizes. Experiments in the laboratory showed that the transformation of graphite to diamond occurred at high pressure and temperature deep within the mantle, although we now know most diamond forms by other reactions (see Winter 2018 Diamonds from the Deep). Subsequently, geoscientists obtained pressure and temperature constraints for diamond formation (from diamond host rocks and their mineral inclusions), bolstering the high-pressure origin for natural diamonds (e.g., Bundy et al., 1961; Mitchell and Crocket, 1971). Evidence of their high-pressure origin meant that diamonds clearly had to have formed before any interaction between kimberlite and crustal rocks such as shale (again, see box B). However, it was still thought that diamonds could crystallize from the kimberlite magma at depth in the mantle before the eruption to Earth’s surface took place, or that diamonds grew under metastable conditions during kimberlite ascent (Mitchell and Crocket, 1971).
In the 1970s, scientists used isotopic dating of kimberlitic minerals to determine the first ages of kimberlite eruptions. Using Rb-Sr geochronology of kimberlitic micas, geoscientists at the University of the Witwatersrand determined that kimberlites from the Kimberley area erupted about 86 million years ago (Allsopp and Barrett, 1975). Around the same time, U-Pb geochronology on kimberlitic zircons of these same kimberlites showed similar results, that they erupted around 90 million years ago (Davis et al., 1976). Later analytical work refined these ages (e.g., Allsopp and Kramers, 1977; Davis, 1977, 1978; Clement et al., 1979; Kramers and Smith, 1983; Smith, 1983). We now know that the majority of Earth’s kimberlites erupted relatively recently (geologically speaking) between 250 and 50 million years ago (see compilations in Heaman et al., 2003; Jelsma et al., 2009; Tappe et al., 2018).
In the 1980s, Stephen H. Richardson and colleagues at MIT, working on diamonds from the Kimberley mines, found that the diamonds range in age from a billion years to more than three billion years old and that they originated in the lithospheric mantle region below the Kaapvaal craton (Richardson et al., 1984). Since the Kimberley kimberlites erupted only 84 million years ago (Clement et al., 1979), the Richardson et al. study showed definitively that the diamonds had no genetic relationship to the kimberlite. This basic age relationship holds for all other diamondiferous kimberlites. Kimberlite eruptions, then, are just the way that diamonds make their way from depth in the mantle to Earth’s surface. Diamonds are simply the passenger, and kimberlites are their transport.
Why Do Diamonds Survive in Kimberlite During Eruption?
Another wonderful feature of the way kimberlites transport diamonds from great depth is that the diamonds manage to survive. Rough diamonds are often resorbed from their primary octahedral shapes into secondary shapes called dodecahedrons. This is because kimberlites are in the process of dissolving the diamond—it’s just that this process has not gone to completion. Nearly all other magmas on Earth, such as basalts and andesites, would completely dissolve diamond, so it is a gift of nature that kimberlites allow diamonds to survive.
Successful diamond transport and delivery also occurs because kimberlites erupt faster and are less oxidizing than other magmas on Earth. Diamonds may also be shielded in pieces of their host rocks during much of their transport. Speed is of the essence here: A low-viscosity kimberlite is estimated to travel at speeds around 8 to 40 miles per hour (Sparks et al., 2006), whereas a normal-viscosity basaltic magma moves at a fraction of this pace. Chemical composition of the kimberlite and its volatile components are also thought to be important factors.
Kimberlite Eruptions in Earth’s History
From field observations made at the site of emplaced kimberlites, kimberlites are more explosive than the eruptions we see today in places like Hawaii, Iceland, Indonesia, and Mount St. Helens. Evidence for crystal granulation, xenolith rounding, and fragmentation (see box A, figure A-1) leads geologists to conclude that kimberlite eruptions are much more violent and breach the surface with the highest velocities of any volcano.
The last known kimberlite eruptions were the circa 10,000-year-old Igwisi Hills kimberlites (Brown et al., 2012) in Tanzania, although there is some debate about whether these constitute true kimberlite. Furthermore, these kimberlites are not diamond-bearing. The next youngest African kimberlites are the 32-million-year-old Kundelungu kimberlites in the Democratic Republic of Congo (Batumike et al., 2008). The most recent diamond-bearing kimberlite-like eruptions were the West Kimberley lamproites (box A), which erupted 24 to 19 million years ago (Allsopp et al., 1985). Around 45% of these lamproites are diamond-bearing, although only two have been mined for their diamonds.
Kimberlites have been erupting since at least the Archean, and the oldest ones discovered so far are the Mitzic kimberlites in Gabon (West Africa), which erupted around 2.8 billion years ago (de Wit et al., 2016). However, kimberlites have not been continuously erupting since that time, and globally there have been several time periods when kimberlites erupted more frequently (Heaman et al., 2003; Jelsma et al., 2009):
Time period (millions of years ago) | 1200–1075 | 600–500 | 400–350 | 250–50 |
% of global kimberlites (from Tappe et al., 2018) | 9.4% | 7.4% | 5% | 62.5% |
How and Why Do Kimberlites Form?
Melt Composition.The primary (or original) melt composition of kimberlite is poorly known because the rock we see today is such a variable, complicated physical mixture. Kimberlite contains magma that has been mixed with many components picked up along the >150 km path to the surface. At the surface, kimberlite contains fine-grained matrix material and minerals known as phenocrysts, foreign minerals known as xenocrysts (diamond being the xenocryst that we want!), and foreign rocks known as xenoliths. In other words, kimberlite is considered a “hybrid” rock. Xenoliths themselves are very interesting to geologists because they are samples of the rock through which the kimberlite has passed.
The predominant mineral in kimberlite is olivine, which could be either phenocrystic (from the kimberlite itself) or xenocrystic (from the mantle and broken off and sampled by the eruption). Making the distinction between these two populations of olivine is not always clear. Olivine is easily altered to a mineral called serpentine, and this alteration also makes estimation of the original magma composition difficult.
There are many different ways to try to determine the primary melt composition: conducting experiments at high pressures and temperatures, looking at melt inclusions found in kimberlite minerals, and performing mass balance calculations where the xenocryst and alteration material are subtracted to arrive at the remaining kimberlite material. All these different approaches now seem to suggest that kimberlite magmas form as melts that are rich in carbonate in the asthenospheric mantle (Stone and Luth, 2016; Bussweiler et al., 2016; Stamm and Schmidt, 2017; Soltys et al., 2018; Howarth and Buttner, 2019). Kimberlite magma forms after low amounts of melting of peridotite (see Winter 2018 Diamonds from the Deep for more information on peridotite), at depths around 200–300 km, and contains high amounts of carbon dioxide and water. The presence of these so-called volatile components in the kimberlite magma is one reason why kimberlite eruptions are thought to be particularly explosive.
Why Did Melting Start? We know now roughly where in Earth kimberlite magmas originated, but why did melting actually start? The “triggers” for deep Earth melting that precede kimberlite eruption are not the same for all kimberlites, and there are three main large-scale geologic scenarios that geologists typically consider:
- mantle plumes rising up from the deep in the mantle and interacting with the cratonic lithosphere
- subduction of oceanic crust and associated collisional processes during supercontinent formation
- tectonothermal events associated with supercontinent breakup (e.g., Heaman and Kjarsgaard, 2000, Heaman et al., 2004; Jelsma et al., 2009; Kjarsgaard et al., 2017)
In particular, rifting of continents and supercontinent breakup—with associated fracturing and brittle deformation in the lithosphere—provide the pathways for kimberlite magmas to reach the surface (e.g., Jelsma et al., 2009). But underlying all these processes of magma generation and the resulting kimberlite eruption is the relationship to the process of plate tectonics. Without plate tectonics to recycle carbonate and volatiles into the mantle, there would be no kimberlites.
Where Do Kimberlites Occur?
Kimberlites do not erupt in all areas of Earth. Globally, kimberlites all occur below the oldest parts of continents, known as cratons (figure 2) (Clifford, 1966; Shirey and Shigley, 2013). Cratons have thick lithospheric roots that extend down to at least 150–200 km, and kimberlite generation in the mantle is probably associated with the physical barrier to mantle upwelling provided by these deep continental roots. Regardless of how kimberlites form, the association of these eruptions with deep continental roots is another of the wonderful mysteries about how kimberlites deliver diamonds. These deep continental roots are Earth’s diamond storehouse.
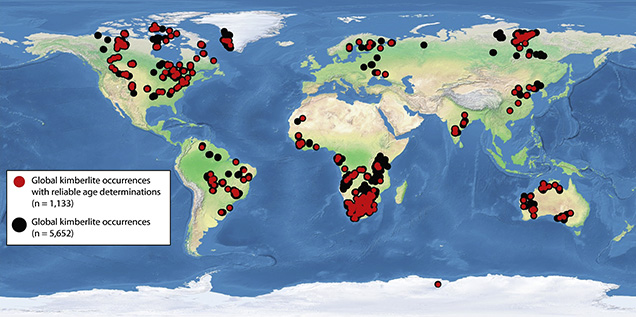
Ongoing Research
There is still much to learn about kimberlites and the relationship between kimberlite magmas and the diamonds that they carry: Why exactly does diamond survive in a kimberlite eruption? What surface features on a diamond are related to the effects of the kimberlite magma versus those that might be caused by fluids deep within the mantle where the diamonds reside (e.g., Fedortchouk, 2019)?
While each kimberlite is unique, general eruption and emplacement models (see box C) are needed to help understand why many kimberlites are devoid of diamonds—is this simply because they did not erupt through diamond-bearing mantle? Or is the lack of diamonds somehow related to dissolution and/or eruption mechanisms? Information such as this is important during exploration and evaluation of new diamond occurrences.
Ultimately there are reasons to care about kimberlites that do not directly relate to their sampling of diamonds but rather to the large-scale view of the solid Earth’s deepest geochemical cycles. Kimberlite magma is an extreme end member for small amounts of mantle melting and high volatile (including water and carbon dioxide) content. How do such melts form and migrate at such high pressures and temperatures? What does the high percentage of young kimberlites reveal about plate tectonics and deep recycling of volatiles? What can kimberlites tell us about the connection between the dynamics of the solid Earth and our major atmospheric greenhouse gas, carbon dioxide?
We have highlighted the basic geological, historical, and practical features of kimberlites. What’s exceptional is that in the end, when a natural diamond is purchased, we have a kimberlite to thank for bringing it to us.