The Lengthy Vertical Journey of Superdeep Diamonds
Aside from their dazzling gem appeal, one thing that makes diamonds so interesting is the great depths below the earth’s surface at which they form. The majority of diamonds mined as gemstones were formed in the base of ancient thick regions of continents, at a depth of about 150–200 km. This is already an incredible depth compared to virtually all the other rocks and minerals around us at the earth’s surface. Even more extreme, however, are superdeep or sublithospheric diamonds, which originate from a depth of approximately 300–800 km (Shirey et al., 2024). With these superdeep origins in mind, how is it that they can be found at the earth’s surface?
Exactly how superdeep diamonds make their way up to the surface has long remained a curiosity. It has been considered that kimberlites and related mantle-source volcanic eruptions might simply originate from comparable depths as superdeep diamonds, providing a single mechanism to carry superdeep diamonds all the way to the surface (Giuliani and Pearson, 2019). The depth of kimberlite formation is still unclear, however, and a shallower kimberlite origin from just below the continental lithosphere, no deeper than 300 km in the asthenosphere, appears more likely (Giuliani et al., 2023).
Such a shallow origin is supported by a recent model that links the timing and placement of kimberlites with convective instabilities or eddy currents associated with continental rifting (Gernon et al., 2023). As illustrated in figure 1, this model places the origin of kimberlites far closer to the surface than superdeep diamonds. An intermediate transport mechanism likely brings them up closer to the base of the lithosphere to be sampled by kimberlites alongside shallower lithospheric diamonds.
Several potential intermediate transport mechanisms have been proposed. These include mantle convection (Davies et al., 2004; Harte, 2010), localized buoyancy of the mantle rocks associated with diamond formation (Smith et al., 2018), upward-percolating melts (Walter et al., 2022), and—perhaps the most widely accepted—mantle plumes, which are hot columns of rock rising through the mantle due to their lower relative density (Stachel et al., 2000; Tappert et al., 2005; Bulanova et al., 2010; Walter et al., 2011; Harte and Richardson, 2012). The timing of this journey with respect to diamond formation and later kimberlite eruption has also been unclear. There may be an intermediate period of storage in the upper mantle (Stachel et al., 2000). A recent study by Timmerman et al. (2023) reporting superdeep diamond ages has shed new light on their lengthy vertical journey.
A PRIMER ON SUPERDEEP DIAMONDS
There are multiple ways in which diamonds can form in the mantle. Diamond growth can occur in different geologic settings and involve different types of host rocks and carbon-bearing fluids (Shirey and Shigley, 2013). Diamonds in the mantle can be broken down into two principal geologic settings or places where they grow: the lithospheric mantle and the sublithospheric mantle (figure 1). Further subdivisions are possible, but for simplicity we will only consider these two overarching groups. Note that here we are strictly speaking about mantle-derived diamonds found in kimberlites and related rocks or their eroded components. These are the kinds of diamonds mined as gemstones, which excludes diamonds from sources such as ultra-high-pressure metamorphic terranes, meteorites, impact sites, and ophiolites.
Diamonds from the lithospheric mantle crystallize in the base of old and thick regions of continental lithosphere (figure 1). These are the most common kinds of diamonds. The second major geologic setting is the sublithospheric mantle, below the rigid lithospheric plates that make up the earth’s surface. Diamonds formed here are termed sublithospheric or superdeep diamonds. Superdeep diamonds make up an estimated 2% of diamonds mined globally, although the exact proportions are not well constrained and can vary greatly by deposit (Stachel et al., 2022).
When we encounter diamonds at the surface, they have been swept up into kimberlites or related mantle-derived igneous rocks. Lithospheric and superdeep diamond populations often mix together, as depicted in figure 1. Mineral inclusions provide a method for identifying these different kinds of diamonds because the mineralogy of mantle rocks changes with depth. When diamonds contain one or more inclusions that could only have been trapped within the sublithospheric mantle, this is conclusive evidence that the host diamond is superdeep. Good examples are ringwoodite (Pearson et al., 2014) or coexisting inclusion pairs of ferropericlase and bridgmanite (found as enstatite) (Stachel et al., 2005).
Superdeep diamonds have been studied with fervor since their discovery unfolded in the mid-1980s (Moore and Gurney, 1985; Scott Smith et al., 1984). A brief history of superdeep diamond research is recounted by Shirey et al. (2024). These crystals obtained from the deep have captivated scientists because they provide the only well-preserved samples of the sublithospheric mantle, making them uniquely suited to study the composition, dynamics, and evolution of the earth’s interior.
More recently, the breadth of superdeep diamonds has increased substantially as two varieties of high-quality gem diamonds were found to be sublithospheric. These are the nitrogen-poor, large, high-clarity diamonds subsequently named CLIPPIR diamonds and type IIb diamonds, which can have beautiful blue colors resulting from their boron content (Smith et al., 2016, 2017, 2018). Both of these newly recognized superdeep varieties, as well as many previously documented diamonds from the Juína region of Brazil and other global localities, have an association with subducted slabs of oceanic lithosphere (Walter et al., 2011; Smith et al., 2021; Regier et al., 2023). Subduction is a major geological process capable of recycling material such as carbon and water from the surface down into the mantle. The fact that superdeep diamonds offer insight into subduction processes makes them all the more valuable for learning about the geological evolution of our planet. For further background on superdeep diamonds, see reviews by Harte (2010), Harte and Hudson (2013), Kaminsky (2012), Shirey et al. (2024), Smith and Nestola (2021), Stachel et al. (2005), and Walter et al. (2022).
AGES OF SUPERDEEP DIAMONDS
Rock and mineral ages help unravel sequences of geologic events. For diamonds, however, measuring an age of crystallization is often difficult and sometimes impossible because it relies on radiometric dating of inclusions. In order to obtain a reliable age, it is essential to obtain a diamond with the right kinds of inclusions to be analyzed for radiogenic isotopes. Radioactive isotopes decay at a prescribed rate and can be used as a sort of internal clock to date inclusions. Generally, the age of an inclusion is assumed to correspond to the age of its entrapment in the diamond host, and therefore the age of diamond growth. Overviews of diamond dating were contributed by Smit and Shirey (2019) and Smit et al. (2022).
Finding superdeep diamonds with the right kinds of inclusions has been challenging, in part because of their rarity and the often small sizes of the inclusions (Shirey et al., 2024). There are fewer diamonds and fewer inclusions to choose from. Another challenge stems from the fact that isotopic measurements need to be representative of the entire inclusion. This is not a problem if an inclusion is homogeneous, but if it is unmixed or separated into multiple phases, the entire inclusion needs to be sampled in a bulk analysis. The latter is typical for superdeep diamonds because their inclusions tend to be retrograded or unmixed in texturally complex mineral assemblages and surrounded by large fractures into which parts of the inclusion may spread. In some cases the large fractures extend to the diamond’s exterior, which effectively opens the inclusion system to leakage or contamination and compromises the inclusion entirely. Some limited preliminary dating work suggested generally young ages compared to lithospheric diamonds (Bulanova et al., 2010; Harte and Richardson, 2012).
Despite these difficulties, a recent study by Timmerman et al. (2023) has found coherent ages from four isotope systems (Rb-Sr, Sm-Nd, U-Pb, and Re-Os) applied to a suite of 11 diamonds from the Juína area of Brazil and two from Kankan, Guinea. Both localities are well-known occurrences for superdeep diamonds. The analyzed inclusions were calcium silicates, interpreted as former calcium silicate perovskite, as well as one example of iron sulfide. Taking all the data together gives overlapping ages of approximately 450 to 650 million years old (Timmerman et al., 2023). Although this range may seem imprecise, it is a remarkable result given the agreement across multiple isotope systems. Establishing this two-locality superdeep diamond age is a big piece of the puzzle of how the diamonds reached the surface.
PUTTING THE PIECES TOGETHER
Ages help put the diamonds into a broader geological context. First, we can compare the 450–650 Ma (million years ago) ages of Juína and Kankan superdeep diamonds with the Cretaceous ages of their host kimberlite eruptions (the Cretaceous period spanned 145–66 Ma). Some of the Juína diamonds were from the Juína-5 and Collier-4 kimberlites, but the remaining Juína and Kankan diamonds studied by Timmerman et al. were from alluvial deposits, eroded from nearby kimberlites. Therefore, the kimberlite eruption age for all the samples was conservatively bracketed as Cretaceous (Timmerman et al., 2023).
The fact that these diamonds formed 450–650 Ma and later arrived at the surface in kimberlites around 145–66 Ma means the diamonds must have spent more than 300 million years in storage somewhere in the mantle. One scenario could be that the diamonds were formed and stored deep in the sublithospheric mantle, perhaps drifting with mantle convection currents. Later, in the Cretaceous, random packages of diamonds of coincidentally similar ages were carried upward by unidentified mechanisms and sampled by kimberlites at Juína and Kankan. However, this scenario of deep and mobile storage does not fit well with the spatial context of these two localities, considering past tectonic plate movements.
At the time of diamond formation, Juína and Kankan, now separated by the Atlantic Ocean, were actually close neighbors in the supercontinent Gondwana. Given that these localities were adjacent, Timmerman et al. suggest that the similar age of the diamonds is not merely a coincidence but an indication that they formed in broadly the same sublithospheric setting. Subducting oceanic lithosphere around Gondwana’s edges (figure 2, step 1) would have made the sublithospheric mantle beneath Gondwana an ideal place for superdeep diamond growth. It is plausible that the studied diamonds formed in the sublithospheric mantle directly beneath the ancient neighboring Juína and Kankan sources (figure 2, step 2).
Since 450 Ma, the Juína and Kankan locations migrated about 6,500 km as the tectonic plates drifted. At the beginning of the Cretaceous, Juína and Kankan separated as the Atlantic Ocean spread open (Timmerman et al., 2023). The notion that subsequent kimberlite eruptions contained those ancient sub-Gondwanan superdeep diamonds suggests that the diamonds were somehow pinned to the overlying continental blocks and were able to migrate with them. Timmerman et al. argue that the diamonds ascended in packages of buoyant, low-density rock shortly following their growth and that these diamond-studded rocks adhered to the underside of the continental lithosphere (figure 2, step 3). Melt-depleted metaperidotitic rock in the subducting slab could heat up near the top of the lower mantle (660 km), becoming less dense than the surrounding mantle and tearing apart to shed buoyant blobs of rock.
It is not an obvious answer that emerges, but with the above lines of reasoning from Timmerman et al. we arrive at the multistage model shown in figure 2. Knowing the diamond ages and being able to put them into the context of subduction and plate movements suggests steps 1 through 4 are successive and related. Subduction beneath Gondwana (step 1) creates a favorable setting for diamond growth (step 2). Portions of the subducted slab heat up and become buoyant, ascending through the mantle (step 3) and adhering to the bottom of the overlying continental lithosphere (step 4). These steps likely occurred within 450–650 Ma, after which the diamonds spent more than 300 million years in storage at the base of the continental lithosphere as the plates drifted across the earth’s surface. At the start of the Cretaceous, continental rifting separates the continental blocks as the Atlantic Ocean opens up (step 5). Mantle instabilities caused by rifting lead to kimberlite eruptions within tens of millions of years (Gernon et al., 2023) and the dormant superdeep diamonds are finally swept up to the surface (step 6).
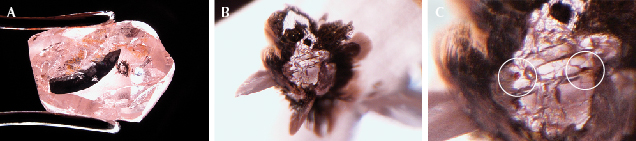
The model outlined in figure 2, with consecutive processes of diamond formation and ascent that are both linked to the subducted slab, could apply to other superdeep diamonds, not just those at Juína and Kankan. An intermediate period of storage in the upper mantle has been proposed previously based on the textures of unmixed or retrograded mineral inclusions (Stachel et al., 2000). For example, some superdeep diamonds contain two-phase inclusions of breyite (CaSiO3) and perovskite (CaTiO3), which are interpreted as unmixed from an original single-phase Ca(Si,Ti)O3-perovskite (figure 3). Unmixing of these two phases would have occurred at a depth shallower than about 300 km, consistent with a period of shallow mantle storage prior to kimberlite eruption (e.g., following step 4 in figure 2). These and other types of inclusions, such as majoritic garnets, show consistent evidence of re-equilibration that could occur during storage in the upper mantle (Timmerman et al., 2023).
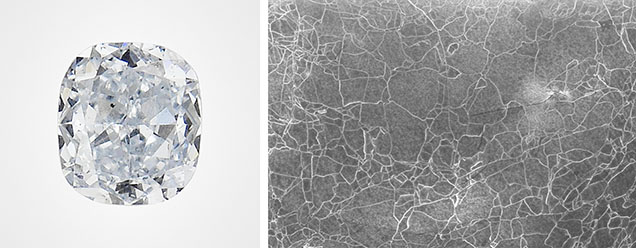
Even beyond mineral inclusions, CLIPPIR and type IIb superdeep diamonds have textural evidence consistent with a period of storage in the mantle. These diamonds contain ubiquitous dislocation networks, seen in cathodoluminescence and deep-UV (<230 nm) imaging (figure 4) (Smith et al., 2017, 2018; Regier et al., 2023). Plastic deformation generates the dislocations, but their movement and reorganization into network patterns requires a period of recovery at high temperatures (Hanley et al., 1977). If these kinds of superdeep diamonds also ascend with buoyant slab-derived rocks and adhere to the continental base, this period of quiescent storage could provide the right conditions for dislocation network formation.
IMPORTANCE FOR DIAMOND MINING AND EXPLORATION
Superdeep diamonds have long been thought of as small and generally not of gem quality, and thus they were deemed irrelevant in assessing the value of a potential diamond deposit. But the recognition that some of the highest-quality and most valuable type IIa and type IIb diamonds are superdeep is changing this perception (Smith et al., 2016, 2017, 2018). Now we can recognize that some diamond mines, such as Letšeng (Lesotho), Cullinan (South Africa), and Karowe (Botswana), derive a major portion of their revenue from superdeep diamonds.
The development of tools for exploration and mining that can specifically target superdeep diamonds would be advantageous. Currently, lithospheric mantle indicator minerals such as eclogitic and chromium-pyrope garnets are used as a proxy for diamond potential, but this tool is blind to superdeep diamonds. Developing a better understanding of how superdeep diamonds reach the surface does not necessarily reveal a simple solution for their exploration, but it might help geologists look in the right direction. It appears likely that superdeep diamonds ascend in packages of buoyant rock and are stored in the upper mantle, which could be an important clue. Host rocks in this intermediate setting might shed distinct indicator minerals or geochemical signatures into erupting kimberlites that are more widespread and easier to detect than the superdeep diamonds themselves.