Plastic Deformation: How and Why Are Most Diamonds Slightly Distorted?
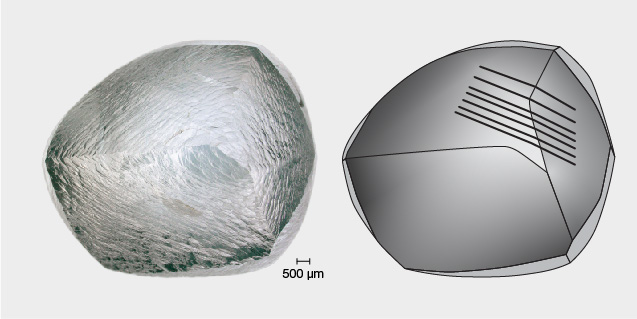
Extreme hardness is one of the most well-known physical properties of diamond. It is difficult to imagine this same material bending or squishing like putty, but many natural diamonds have evidence of plastic deformation (figure 1). This process is even responsible for creating the value behind the most expensive diamonds sold at auction to date, by both per-carat price and total price. Specifically, the 11.15 ct Williamson Pink Star that sold for $57.7 million and the 59.6 ct Pink Star that sold for $71.2 million owe their Fancy Vivid pink colors to plastic deformation.
Plastic deformation does not always lead to pink color, though. More commonly the result is brown color. Our understanding of brown color in diamond has improved in the last 20 years, in part due to the need to screen for decolorizing high-pressure, high-temperature (HPHT) treatment. Most mined diamonds have at least some amount of brown color due to plastic deformation, varying from dark to barely noticeable (Harris et al., 1979; Fisher, 2009; Dobrinets et al., 2013). In other instances, where the deformation is less intense, there may be no color imparted. Plastic deformation is one of the most prevalent features in natural diamond (Urusovskaya and Orlov, 1964; Harris et al., 1979). It is a natural phenomenon thought to occur sometime after a diamond crystallizes, during its residence deep in Earth’s mantle and/or during its volcanic journey up to the surface.
WHAT IS PLASTIC DEFORMATION?
When stresses act on a solid, they can cause it to deform, or change shape, in one of three ways. There is elastic deformation, the kind of deformation that springs are designed for. When you remove the stress, it “springs” right back into its original shape. Brittle deformation is the kind of deformation at play when a water glass falls to the floor and shatters. The material fractures and breaks apart. Finally, there is plastic deformation, the kind of deformation associated with putty. The material deforms into a new shape but the change is lasting, even after removing the stress.
A familiar wire paper clip can serve to demonstrate deformation. If you bend it just slightly, it will deform elastically and return to its unbent shape. The elastic behavior is what allows a paper clip to hold on to a few sheets of paper. If you bend it beyond a certain point, the bend becomes permanent. This is plastic deformation. If you bend it back and forth a few times in one spot, the paper clip will break.
Often we see just one of these three mechanisms dominate, whether it is elastic, brittle, or plastic. Deformation behavior depends on many factors, such as the temperature, how much stress is applied, and, of course, what kind of material it is. For diamond, temperature is a key factor. If you tried to squeeze, twist, or stretch a diamond at room temperature, the elastic deformation would be minimal. It is among the most rigid of all known materials. If it is subjected to high stresses, diamond tends to exhibit brittle deformation by cracking or chipping. But at higher temperatures, diamond begins to exhibit plastic deformation. Experiments show that significant plastic deformation requires temperatures above approximately 900°C (DeVries, 1975; Weidner et al., 1994).
HOW DOES DIAMOND DEFORM PLASTICALLY?
Many metal objects, including paper clips, deform plastically under conditions we can easily create and deform to a degree that is obvious. Our understanding of how crystalline solids deform is underpinned by engineering studies of metals, such as steel used for car frames and bridges. Aluminum foil is an example of a metal whose plastic deformation behavior can be felt as we shape and conform it in the kitchen. Although we cannot feel and observe diamond deformation in the same way, it involves the same basic principles at the atomic scale. During plastic deformation, atomic bonds are breaking and re-forming along imperfections called dislocations. Plastic deformation in all crystalline solids, including diamond, is accomplished by the creation and movement of dislocations (Nesse, 2017).
A dislocation is a linear (one-dimensional) disruption in the regular, repeating atomic structure. Figure 2 shows a simplified illustration of a dislocation in a crystal lattice. The dislocation is shown end-on, and the crystal lattice appears to have an extra half-plane of atoms, shown in orange (figure 2), which resembles a half sheet of paper inserted into an otherwise orderly paper stack. This is an edge dislocation, one of two major dislocation types. The second type is a screw dislocation, which has a different geometry.
Dislocations allow a crystal to deform plastically little by little as bonds break and re-form. The crystal as a whole remains fully intact, as only a small number of its bonds are broken at any given time. A dislocation is much like a wrinkle in a carpet (figure 2, bottom). A carpet could be moved by introducing a wrinkle and working it across the carpet. Most of the carpet remains in contact with the floor, but moving the wrinkle shifts the carpet slightly by breaking and reestablishing contact with the floor. Similarly, as dislocations move through a crystal, they can allow atomic layers to move with respect to one another. Systematic movement of many dislocations can allow the whole crystal to deform plastically when subjected to stress.
Given that crystals are orderly structures, it should come as no surprise that dislocation movement is not random. The crystal structure constrains the dislocation movement into certain configurations and directions. In figure 2, dislocation movement or “slip” occurs along a plane (shown as a blue dashed line) that is parallel to the atomic layering. In diamond, slip occurs along octahedral or {111} planes, with the direction of slip within those planes being described by <110>, together giving a “slip system” described as {111} <110> (Evans and Wild, 1965). In other words, when diamond deforms, the deformation takes place along internal {111} planes, which is the same orientation as the faces of an octahedron-shaped diamond crystal. And the three lines defining the edges of that triangular octahedral face have a <110> orientation, which illustrates the three possible directions of slip within that octahedral plane. Slip planes will contain many dislocations, not just one, and deformation can be spread across multiple dislocations and multiple slip planes simultaneously.
WHAT DOES IT LOOK LIKE?
Plastic deformation involves the creation and movement of dislocations through the diamond crystal lattice. Even though this is something that happens at the atomic scale, it does leave some visible signs. Figure 1 shows plastic deformation lines as a series of fine parallel ridges on a diamond’s surface. These lines trace out where the internal {111} slip planes meet the outer surface of the diamond. They stand out because the diamond surface is resorbed, meaning it has been partially dissolved or etched by natural fluid or magma (Smit and Shirey, 2020). The slip planes do not etch at the same rate as neighboring regions of the crystal, resulting in surface relief and lines visible to the unaided eye.

Plastic deformation lines (figure 1) are the archetypal feature of plastically deformed diamonds (Harris et al., 2022). They are frequently but variably observed within some diamonds from most diamond deposits (Harris et al., 1979). However, only diamonds with resorbed surfaces can show deformation lines (Gurney, 1989). On primary octahedral crystal faces, evidence of plastic deformation can occasionally be observed as rows of trigons (figure 3). These negative trigons are small pits where the diamond has been etched away preferentially due to the presence of dislocations. Each row of trigons marks a separate {111} plane.
In addition to dislocation movement on {111} planes, a related plastic deformation mechanism can lead to the crystal lattice being distorted into a totally new orientation. This abrupt and localized change in crystal lattice orientation is called mechanical twinning or deformation twinning. Unlike twins arising from growth, such as macles (Harris et al., 2022), mechanical twins in diamond are strictly from deformation (Titkov et al., 2012; Yu et al., 2012). This process is sometimes called glide, and the resulting mechanical twin planes are called glide planes (Nesse, 2017). In fact, the rows of trigons in figure 3 technically reveal glide planes, which in this case are associated with a pink color. The close-up (figure 3, right) shows that the trigons are connected by thin pink lines, actually pink lamellae viewed end-on, that correspond to glide planes. Pink lamellae like these are typically about 1 micrometer thick and may consist of multiple closely spaced mechanical twins (Gaillou et al., 2010).
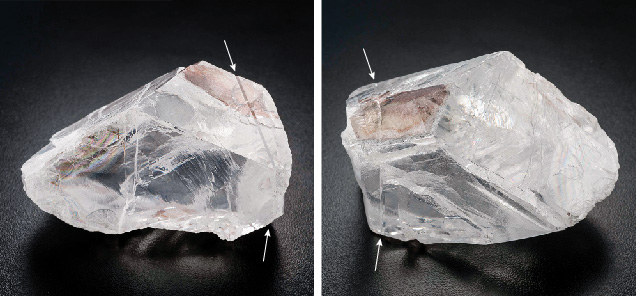
A more dramatic example of mechanical twinning is shown in figure 4. A sharp step in surface topography runs all the way around this large rough diamond like a belt and perfectly traces out a {111} plane. This is a ~0.5 mm thick mechanical twin zone, where the diamond has been distorted and the lattice reoriented as a result of applied stress. Again, the new lattice orientation is not random, but rather a symmetrical mirror image so that the different lattice orientations match up neatly at the interface between the twinned region and the rest of the diamond on either side. Natural broken cleavage surfaces intersecting the mechanical twin (figure 4, left) demonstrate the change in crystal orientation by a corresponding change in the cleavage direction within the ~0.5 mm thick twinned zone.

Rough diamonds and their naturally sculpted surfaces can reveal a lot about their deformation history. Thankfully, since plastic deformation happens internally, it is still possible to observe it once a diamond is cut and polished. Polarizing filters are a simple yet powerful tool for this application, whether using a polariscope or a gem microscope (Renfro, 2015). Viewing a diamond using crossed polarizing filters is an easy way to see anomalous birefringence, which in natural diamond is typically the result of plastic deformation (figure 5). With this technique, a perfect, unstrained diamond should appear uniformly dark inside. Bending and twisting of the light as it passes through deformed (strained) regions of the diamond will result in a pattern of anomalous birefringence, such as banding, mottling, or cross-hatching.

Plastic deformation can also be observed using cathodoluminescence imaging or deep UV fluorescence imaging (e.g., DiamondView). These techniques are especially useful for examining polished diamond surfaces, where slip planes can appear as bright or dark lines (figure 6, left). Another deformation-related pattern sometimes revealed with these methods is dislocation networks (figure 6, right), which have a cellular appearance resembling mud cracks or a fine mesh (De Corte et al., 2006). Dislocation networks are thought to be the result of not just plastic deformation, but also a period of recovery at high temperatures that allows the dislocations to reorganize into a more stable configuration (Hanley et al., 1977). This process may be similar to hammering or working metals and then heating them up to relax and reconfigure the tangled dislocations that have been introduced. Sublithospheric or superdeep diamonds often have dislocation network patterns, and it has been suggested that their formation is promoted by low nitrogen content and/or high temperatures (Smith et al., 2019).
HOW DOES PLASTIC DEFORMATION CAUSE COLOR?
In 1999, General Electric and Lazare Kaplan International announced that HPHT processing could be applied as a commercial treatment to decolorize brown diamonds (Vagarali et al., 2004). This spurred research into the cause of brown color, which up until that point had been poorly understood. Visual indications of deformation had long been recognized to correlate with the presence of brown and to a lesser extent pink colors in diamond (Urusovskaya and Orlov, 1964; Harris et al., 1979). Internally, these colors often concentrate along slip planes, glide planes, or less distinctly along “graining,” all of which are deformation features. Theoretical modeling and experiments played important roles in linking brown color to dislocation movement and the creation of vacancies in the crystal structure (Avalos and Dannefaer, 2003; Hounsome et al., 2006; Barnes et al., 2007).
A vacancy is a missing atom in the crystal lattice. Vacancies can be created during plastic deformation when multiple dislocations interact or jogged dislocations move through the crystal (Leipner et al., 2000, 2003). At the high temperatures necessary for plastic behavior, these vacancies are mobile and tend to cluster into groups. These small groups of vacancies can absorb visible light and cause color. Vacancy clusters made up of about 40−60 vacancies are thought to be responsible for the common brown color in diamond (Avalos and Dannefaer, 2003; Hounsome et al., 2006; Barnes et al., 2007).
The cause of deformation-related pink is believed to be similar but involves a broad absorption band centered near 550 nm that is not fully understood (Gaillou et al., 2010). To be fair, not all brown and pink color is attributable to plastic deformation, but it is the leading cause (Harris et al., 1979; Fisher, 2009). An excellent review of brown and pink color in diamond was presented in Eaton-Magaña et al. (2018).
WHAT CAUSES THE DEFORMATION?
It is plain to see that many natural diamonds bear evidence of plastic deformation, but it is not intuitively obvious why this should be the case. There must be some geological processes that lead to compression or shearing forces that occasionally deform diamonds. The geological process should be neither too gentle, because the relatively soft silicate host rocks should simply flow around the diamonds, nor too violent, which would break diamonds rather than plastically deform them. Several theories have been proposed.
Kimberlites and related eruptions that bring diamonds from the mantle up to the surface could have the power to deform diamonds. The magmatic journey is thought to be relatively violent, however, and it is clear that diamonds can break as they ascend within a kimberlite (Harris et al., 2022). This may mean that the conditions are too forceful and chaotic to develop significant plastic deformation. The early stages leading up to an eruption could also provide opportunities for diamond deformation.
Another possibility is that diamond deformation is related to the movements at the lithosphere-asthenosphere boundary, where the base of a rigid continental tectonic plate meets underlying weak, mobile rocks at a depth of approximately 200 km (Stachel et al., 2018). This would involve shearing between the lithosphere, the rigid host rock where most diamonds form, and the asthenosphere, the underlying warm flowing rock that is the topmost part of the convecting mantle.
Yet another theory suggests that deformation in some settings might be related to the movement of tectonic plates, specifically oceanic lithosphere sinking into the mantle by the process of subduction (Bulanova et al., 2018). Subduction and the motion of tectonic plates could also play a role in the deformation of superdeep diamonds. The formation of these diamonds has been linked to deep-focus earthquakes (Shirey et al., 2021), which could involve localized shearing and faulting, potentially favorable conditions for plastic deformation of diamonds.
The deformation histories recorded within natural diamond are valuable for studying Earth’s interior. In the future, it may be possible to constrain the temperature, pressure, and strain rate (i.e., the speed of deformation) required to produce the various features we observe. This information about deep mantle processes would help paint a clearer picture of how and why rocks deep inside the earth move around over geologic time.
DIAMONDS BEAR WITNESS TO OUR ACTIVE PLANET
The fact that natural diamonds frequently contain evidence of deformation reminds us that Earth is not a static ball of rock. As far as we know, Earth is the only planet with active plate tectonics, meaning its outer layer is made up of multiple moving plates (Condie and Pease, 2008). The processes of mantle convection and subduction mean Earth’s interior is on the move as well. Measurable effects of these mechanisms exist in the chemical and isotopic composition of diamonds and their inclusions. But what makes a diamond’s plastic deformation features special is that they are a visible, tangible testament to rock and magma churning, shearing, and moving about inside our active planet.