Gemological Characterization of Montana Sapphire from the Secondary Deposits at Rock Creek, Missouri River, and Dry Cottonwood Creek
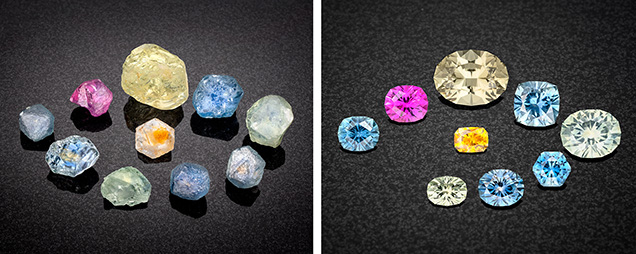
ABSTRACT
Montana has produced large volumes of sapphire from secondary deposits for more than 150 years. While early production was mostly for industrial use as watch and instrument bearings, modern heat treatment technology has enabled the deposits to be worked profitably as gemstone mines, bringing more of this material to the gem and jewelry trade than ever before. Although pale blue and pale green sapphires make up the bulk of the production, fine Montana sapphire comes in nearly every hue, including yellow, orange, purple, and pink, along with very rare finds of ruby. Secondary Montana sapphire shares some similarities with those from Umba and Songea in Tanzania, but it is generally straightforward to separate them on the basis of inclusion scenes and trace element chemistry fingerprints.
Nearly 160 years ago, gold prospectors in the American West stumbled upon one of North America’s most important gemstone deposits, discovering sapphire in several locations in Montana. These were first recovered along the Missouri River near Helena, and additional deposits were later found at Rock Creek and Dry Cottonwood Creek. The sapphires recovered from these secondary deposits generally have overlapping properties and are collectively referred to as “Montana sapphire.” [Note that these are distinct in their gemological properties from “Yogo sapphires,” which are mined from Montana’s only primary deposit, at Yogo Gulch; see Renfro et al. (2017).] Surprisingly, the secondary deposits were not initially mined for fine jewelry-grade sapphire but for gold and for industrial-grade sapphire to use as watch and instrument bearings.
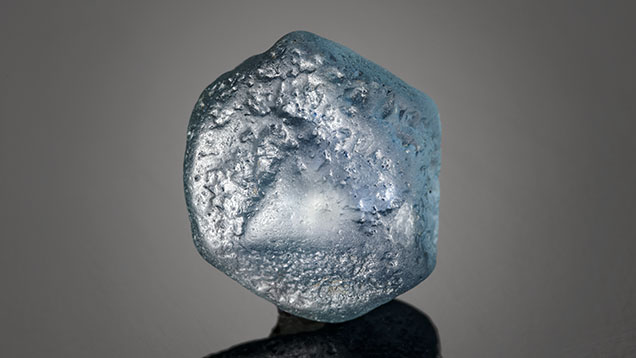
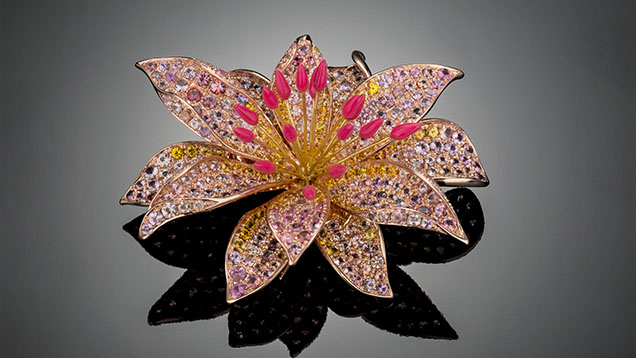
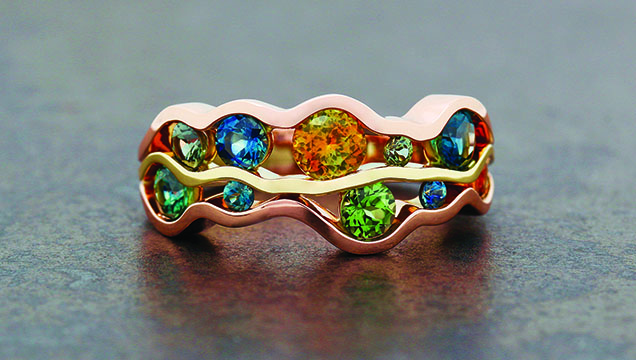
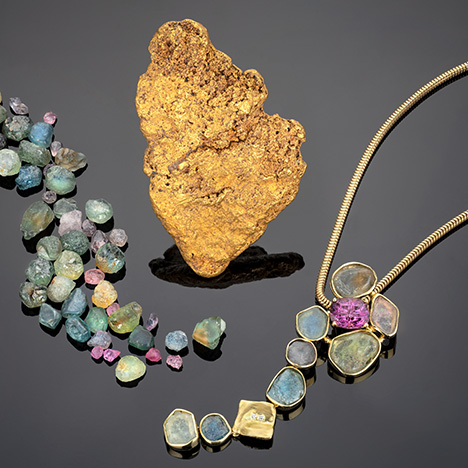
While the deposits produce many stones with exceptional natural color, the majority require (or at least benefit from) carefully controlled high-temperature heat treatment to enhance their color for the jewelry market. Various heating procedures—in both oxidizing and reducing environments at high temperatures—can be used to either create or intensify a variety of colors (e.g., Emmett and Douthit, 1993). Whether heated or with a natural, untreated color, secondary Montana sapphire occurs in a wide range of sizes and colors including blue, green, yellow, orange, purple, and pink (figures 1–5). With the reintroduction of large-scale mining 25–30 years ago and the recent acceleration of mining activities at Missouri River and especially Rock Creek, fine Montana sapphire is available in the gem and jewelry market in quantities never seen before. It is also growing in popularity with the increasing emphasis on transparency and traceability in supply chains within the jewelry industry. Demand is also increasing as consumers become more interested in the less saturated blue and teal and fancy-color sapphires that are common in Montana. Fortunately, these sapphires have unique gemological and chemical characteristics that allow their geographic origin to be confidently determined.
MATERIALS AND METHODS
Samples. The Montana sapphires examined in this study are from a variety of sources. The bulk of the samples were collected in 2015 by GIA gemologists at the Potentate mine at Rock Creek and from Cass Thompson’s mining operation at Eldorado Bar on the Missouri River. Additional samples came from the collections of the authors and were generally sourced directly from current operations at Rock Creek and Missouri River. Sapphires from the South Fork of Dry Cottonwood Creek were donated by Dr. John Emmett, director of Crystal Chemistry in Brush Prairie, Washington, who obtained them from the mining operations of American Gem Corporation in 1994–1995. Observations described in this study come primarily from the more than 800 sapphires in the GIA Colored Stone Reference Collection gathered from the sources described above. Additional sapphires were provided from the collection of John I. Koivula, GIA’s analytical microscopist. The largest sapphire studied was the 67.15 ct Yellowstone sapphire, but most were much smaller, from less than a carat to several carats in size. Seventy-three Montana sapphires were included in our laser ablation–inductively coupled plasma–mass spectrometry (LA-ICP-MS) trace element measurements, as well as 19 sapphires from Songea, Tanzania, and 17 from Umba, Tanzania. The Songea and Umba sapphires were either collected directly in the field by GIA field gemologists or donated by Dr. Emmett.
Ultraviolet/Visible/Near-Infrared (UV-Vis-NIR) Spectroscopy. Spectra were collected with a PerkinElmer Lambda 950 UV-Vis spectrophotometer (PE 950) in the 200–1000 nm range, with 1 nm spectral resolution. Spectra presented were collected from samples oriented as wafers perpendicular to the optic axis, which allows collection only of the ordinary ray (o-ray) spectrum. Samples used in heat treatment experiments were mounted on aluminum plates with 1 mm apertures during spectroscopy. They were set in place using wax to allow the same region of the sample to be measured before and after heat treatment.
Fourier-Transform Infrared (FTIR) Spectroscopy. FTIR spectra were collected using a Thermo Fisher Nicolet 6700 FTIR spectrometer equipped with an XT-KBr beam splitter and a mercury-cadmium-telluride (MCT) detector operating with a 4× beam condenser accessory. The resolution was set at 4 cm–1 with 1.928 cm–1 data spacing.
Raman Spectroscopy. Inclusions were identified, when possible, using Raman spectroscopy with a Renishaw inVia Raman microscope system. The Raman spectra of the inclusions were excited by a Modu-Laser Stellar-REN Ar-ion laser producing highly polarized light at 514 nm and collected at a nominal resolution of 3 cm–1 in the 2000–200 cm–1 range. In many cases, the confocal capabilities of the Raman system allowed inclusions beneath the surface to be analyzed.
LA-ICP-MS. Trace element chemistry was collected by LA-ICP-MS using a Thermo Fisher iCAP Qc ICP-MS, coupled to an Elemental Scientific Lasers NWR 213 laser ablation system with a frequency-quintupled Nd:YAG laser (213 nm wavelength with 4 ns pulse width). Ablation was carried out with 55 µm spot sizes, with a fluence of 8–10 J/cm2 and repetition rates of 20 Hz. The isotope 27Al was used as an internal standard at 529250 ppmw, and synthetic and natural corundum reference materials were used as external standards (Stone-Sundberg et al., 2017). Detection limits ranged from 0.1–0.3 ppma for magnesium, 0.5–2.0 ppma for titanium, 0.03–0.2 ppma for vanadium, 0.06–1.3 ppma for chromium, 1–5 ppma for iron, and 0.03–0.07 ppma for gallium. Trace element values are reported here in parts per million on an atomic basis rather than the more typical parts per million by weight unit used for trace elements in many geochemical studies. Units of ppma are the standard used in GIA laboratories for corundum, as they allow a simpler analysis of crystal chemical properties and an understanding of the color mechanisms of sapphire and ruby. Conversion factors are determined by a simple formula that can be found in table 1 of Emmett et al. (2003).
Heat Treatment. Some heat treatment studies were carried out at the commercial heating facility operated by Dale Siegford of the Sapphire Gallery in Philipsburg, Montana. Treatments were claimed to have been at 1400°C, but other details of this commercial treatment were not disclosed. Additional heat treatment experiments were carried out at GIA’s experimental heating facility using a Thermal Technology Model 1000-3560-FP24 muffle tube furnace, similar to the system used in Emmett and Douthit (1993).
Gemology. Standard gemological instruments were used to measure refractive index, birefringence, specific gravity, and pleochroism. Microscopic observation was performed using either a Nikon Eclipse LV100 compound microscope or Nikon SMZ1500 binocular microscope outfitted with a Nikon DS-Ri2 camera for recording images. Differential interference contrast (DIC) images were taken on the LV100. Also used were a Nikon SMZ25 stereomicroscope equipped with dual fiber-optic illuminators, darkfield and brightfield illumination, polarizing filters, and a Nikon DS-Ri2 camera.
HISTORY
Like much of the American West, the history of Montana is told in the story of gold. The original prospectors who discovered the state’s sapphire deposits generally had little in the way of mineralogical, much less gemological, knowledge. While these prospectors did stumble upon one of the most important gem deposits in the North American continent, they were mainly targeting gold, whose value was much more obvious than the shiny dense pebbles of sapphire they sometimes found in their jigs and pans. When the first sapphires were discovered in 1865 at the Missouri River, their range of natural colors did not suit the gem and jewelry industry of that time—that happened 30 years later, when bright blue sapphires were found at Yogo Gulch. However, when sapphires were found in abundance at Rock Creek in 1892, another lucrative market opened up for these stones as watch and instrument bearings (figure 6). In fact, mining efforts until the late 1920s were driven almost entirely by the search for gold and the use of sapphires as watch bearings. The bulk of the sapphires mined in Montana had ended up being used for industrial purposes. The availability and affordability of synthetic ruby and sapphire (grown by the Verneuil process) in the 1920s and 1930s ended the first great heyday of sapphire mining in Montana (Berg, 2014). After some small-scale mining to support the war effort in the 1940s, the major operations in this early period ended shortly after World War II. It was not until the introduction of advanced heat treatment procedures that these deposits became economically viable again.
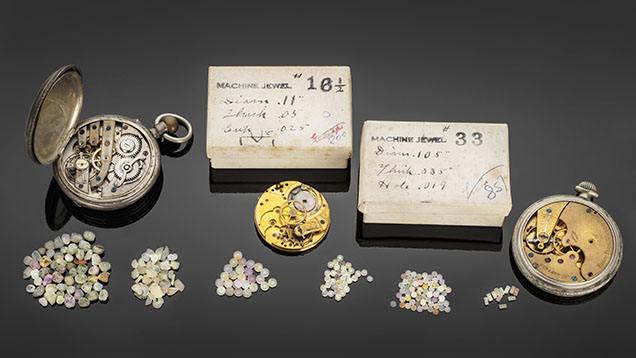
The following sections will document each of the three secondary sapphire deposits in more detail. Historical information in this section comes primarily from the works of Berg (2007, 2014) and Berg and Landry (2018), which are currently the most comprehensive historical works on sapphire mining in these deposits. These three publications contain original historical research based on archived historical resources and interviews with primary historical figures. When possible, the primary published source of information is cited; however, when no citation is provided, it should be understood that the primary reference is the original historical research in Berg (2007), Berg (2014), or Berg and Landry (2018) for Dry Cottonwood Creek, Rock Creek, and Missouri River, respectively. Additional sources of historical information are Kunz (1890), Pratt (1906), Clabaugh (1952), Emmett and Douthit (1993), Garland (2002), Barron and Boyd (2015), Hsu et al. (2017), and Kane (2020).
Missouri River. The first influx of European settlers into the Montana territory began in 1862 with the discovery of rich gold placers in Grasshopper Creek at the town of Bannack. With rumors of gold circulating, prospectors would seek their fortune up and down nearly every creek and river in the territory, no matter how large or small. Gold in abundance would be found the next year at Virginia City in Alder Gulch and, importantly, at Last Chance Gulch near Helena.
The first report of sapphire came in 1865, when gold prospector Ed Collins found several while working a gravel deposit along the Missouri River near Helena at what came to be known as Eldorado Bar. The stones were found not in the river itself, but in gravel beds sitting atop bedrock just off the banks of the river, sometimes several hundred meters above water level. Over the years, similar deposits were identified along a roughly 21 km stretch of the Missouri River, with eight major gravel bars eventually worked to some extent. Collins was apparently unsure what to make of the gemmy stones he found, so he sent them east to Tiffany & Co. in New York and other parties overseas (“Eldorado Bar sapphires,” 1867). The gems were identified as sapphire, although the discovery generated little attention (Browne, 1868, p. 50). These gravel bars sitting alongside the Missouri River were also rich in gold, so mining activities continued regardless.
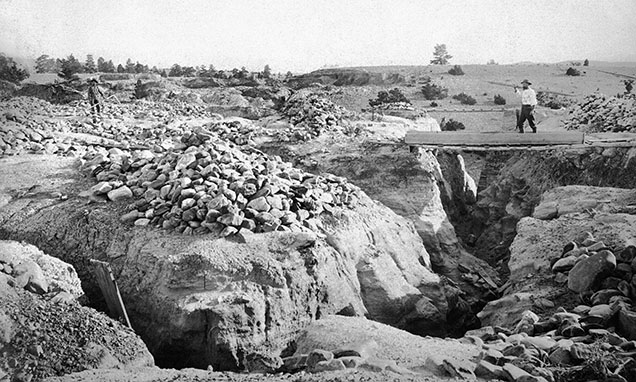
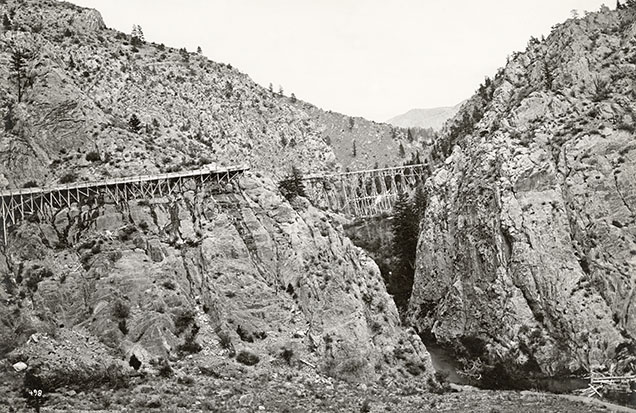
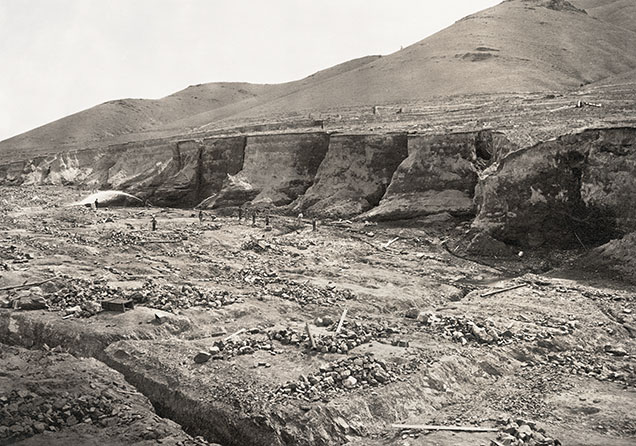
The earliest workings on these bars involved horizontal drifts cut through the gravel beds, which allowed miners to avoid the labor of removing the several meters of overburden that typically overlie the sapphire- and gold-bearing gravels (figure 7). Hydraulic mining, which had been used at other gold camps, would have been more efficient. Yet here, as in much of the American West, this advanced mining technique was limited by access to water. Despite their proximity to the Missouri River, the gravel beds were at a higher elevation than the river level, so the water would have to be pumped up to the gravel beds or brought in from elsewhere. The first major mining efforts commenced in 1868, when the Eldorado Ditch Company completed a ditch from Trout Creek, at a higher elevation than the Missouri River, to bring water to the upper terraces of Eldorado Bar (figure 8). This allowed the gravel beds to be worked by hydraulic mining, in which high-pressure jets of water were employed to wash sapphire-bearing gravels into sluice boxes with riffles set up at intervals to capture sapphire and gold (figure 9). This led to the consolidation of smaller claims on Eldorado Bar, creating larger and more efficient operations. The process of consolidation continued until 1872, when original discoverer Ed Collins joined with seven partners to control a 641 hectare claim encompassing most of the upper terrace of Eldorado Bar.
During a lull in mining in the 1880s, brothers Frank D. and Thomas H. Spratt began buying up and staking new mining claims, especially on Eldorado Bar. In 1887, with the financial backing of a third brother, Augustus N. Spratt, they incorporated the Trout Creek Mining Company and began actively marketing the sapphires on a national and international scale. In 1890, an English syndicate was formed to market Missouri River sapphires. The syndicate ended up purchasing properties on Ruby Bar owned by Frank and Edgar Langdon, as well as properties belonging to the Spratt brothers at French Bar and their Eldorado Bar claims. The English syndicate now controlled most of the profitable workings and was incorporated as the Sapphire and Ruby Company of Montana, Ltd. with initial public stock offerings of 400,000 shares at £1 (“Montana’s precious stones,” 1891; “Sapphire and Ruby Company prospectus,” 1891). Sapphire and gold production commenced swiftly, with drift mining utilized initially and hydraulic mining techniques applied later. The Spratt brothers also incorporated the Spokane Sapphire Company as a subsidiary of the Sapphire and Ruby Company of Montana, Ltd. to mine Spokane Bar.
Given the worldwide interest being generated about the Missouri River deposit, other mining enterprises came along as well. Notably, Henry Matheson acquired claims on Emerald Bar and brought in water with the use of a steam pump. Matheson soon sold out to a group of investors out of Helena forming the Montana Gold and Gem Company.
Despite the budding success of Missouri River sapphires, which were featured at the 1893 Chicago World’s Fair, the economic Panic of 1893 crippled the market for them. This was compounded, in no small part, by the discovery in 1895 of sapphires at Yogo Gulch whose natural cornflower blue color was preferred in the gem market. Shortly thereafter, the Sapphire and Ruby Company of Montana, Ltd. went bankrupt and its properties reverted to Augustus N. Spratt, who reformed his holdings as the Eldorado Gold and Gem Company.
In 1911, the lowest terraces of Eldorado and Spokane Bars were submerged with the completion of the Hauser Dam and the creation of Hauser Lake. While it may seem a pity to flood precious gem-bearing gravels, the advantages of the newly created lake did not go unnoticed. Most notably, in 1938, the Perry-Schroeder Mining Company was formed and purchased a Yuba bucket-line dredge. The Perry-Schroeder Mining Company was one of the few operations allowed to continue mining gold throughout World War II despite War Production Board Order L-208, which shut down gold mining operations across the country in order to focus extraction efforts on strategic materials. The company was only allowed to continue mining gold because it was also producing industrial sapphire, which was in short supply given that the synthetic sapphire manufacturers were all located in Europe at the time. The Yuba dredge continued mining for gold and sapphires until 1947, marking the end of the historic mining activities on the Missouri River. In the ensuing years, several smaller operations continued to mine for sapphire along the gravel bars of the Missouri River. Their efforts have been fueled by the development of high-temperature heat treatment processes to improve the sapphire colors, making them more desirable in the global gem and jewelry market. Today, the mining is nearly all concentrated on Eldorado Bar, where small-scale miners work some virgin gravels but mostly the tailings left over from the Perry-Schroeder Mining Company in the 1940s. As that dredging operation was also tailored to recover gold, the company’s sapphire recovery was fairly low compared to modern operations, and many sapphires were left behind.
Between 1988 and 1993, Sam Speerstra mined six million carats of sapphire from Eldorado Bar (Berg and Landry, 2018). Adjacent to American Gem Corporation’s main lease, Gem Resources Management Corporation mined Eldorado Bar from 1988 to 1992. While American Gem Corporation’s primary mining activities were focused at Rock Creek (see “Rock Creek” history section below), their press release from September 1994 indicated that consulting geologists and engineers had completed bulk sample testing on 260 of the nearly 1,200 acres (105 of 485 ha) at Eldorado Bar controlled by the company (O’Donoghue, 1995). These tests were conducted to determine occurrence and grades (carats per cubic yard) and indicated considerable sapphire reserves at Eldorado Bar. American Gem Corporation also conducted test sampling across the river channel at Dana’s Bar. The company never moved beyond the testing phases, electing instead to concentrate its efforts at Rock Creek and Dry Cottonwood Creek.
Present-day mining includes operations of the Spokane Bar mine and Gold Fever Rock Shop (currently the largest operation), Neal Hurni with Lewis and Clark Sapphires, Blaze Wharton with Blaze-N-Gems, Bruce Scharf and Blue Jewel mine, Don Johnson with Obsessed Over Gems, and Tim Beard along Gruell’s Bar. Another notable operation in the recent past is that of Mac Mader, who constructed a suction dredge in use from 1983 to 1986 to recover gold and sapphire from Hauser Lake. In 2012, Mader used a handheld suction dredge operated from a small barge-mounted washing plant on Hauser Lake near French Bar.
Rock Creek. The discovery of sapphire in the Rock Creek district is often attributed to one Emil Meyer, a German immigrant who later partnered with Swiss native L.J. Moffat to work the deposit (“Sapphire mined on Rock Creek after 1892 discovery there,” 2019). However, some reports attribute the discovery to M.H. Bryan Sr. of Philipsburg, who claimed to have been prospecting for gold on the West Fork of Rock Creek when he noticed several bright stones at the bottom of each pan of dirt. He collected the stones and simply gave the choice gems to his grandchildren to play with. Regardless of who truly discovered the deposit, or whether they found it more or less contemporaneously, the recorded date of discovery is 1892. An article from the September 3, 1892 edition of The Anaconda Standard (“New sapphire fields,” 1892) indicates that local prospectors were already aware of the rich sapphire diggings and were actively preparing to start working the deposit.
While this deposit is colloquially referred to as “Rock Creek,” sapphires have largely been mined from several tributary gulches that cut into the mountain to the north of the West Fork of Rock Creek. The two most important, especially in the early days of mining, were Anaconda Gulch and Sapphire Gulch, but mining has taken place at numerous gulches as well as in placers along the banks of the West Fork of Rock Creek, in an area called the Meadow. Also of particular note for this deposit is the relative lack of gold, especially compared to the Missouri River deposit. What Rock Creek lacks in gold it more than makes up for with an overwhelming abundance of sapphire. Rock Creek has produced more than any other deposit in Montana, including Yogo Gulch, likely eclipsing all of them combined by nearly a factor of 10. All told, at least 70 metric tons of sapphire have been mined from the Rock Creek area (Berg, 2014). However, this estimate does not include the mid-1990s yield of nearly 4 million carats by the American Gem Corporation or significant recent production from Potentate Mining (Kane, 2020). In fact, Rock Creek is the only secondary deposit in Montana to be worked only for its sapphire content (gold is recovered there as well but has never been the primary focus of mining).
The early years saw the deposits worked mostly by smaller operations. Figures of note from the first few years include 400,000 carats of sapphires produced by William Knuth of Helena and William Moffitt of Philipsburg in 1900, of which 25,000 carats were fit for cutting. L.J. Moffat and Emil Meyer were two of the important early miners at Rock Creek. Moffat’s Swiss heritage may have helped position these stones in the industrial sapphire market, as most of the production was used by fine watchmakers in Switzerland. Their importance in the early days of mining was recognized with the naming of sapphire-bearing gulches. Meyer Gulch was a productive tributary of Sapphire Gulch, and Moffat has two gulches named in his honor: the Little Moffat and the Big Moffat.
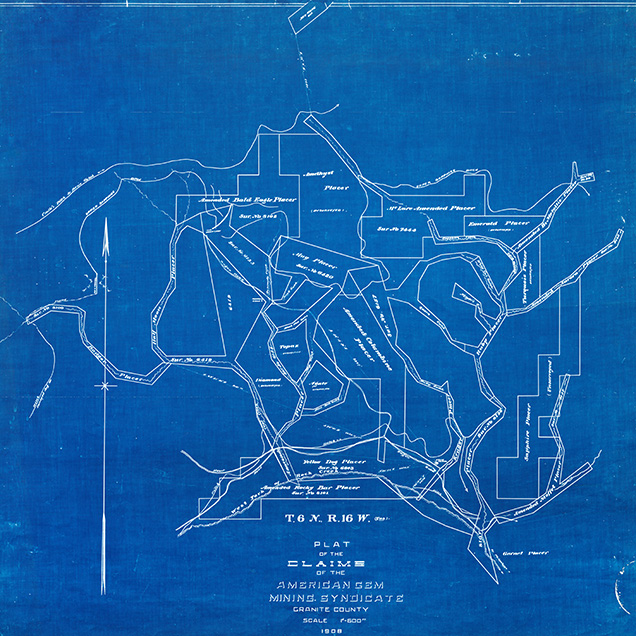
The most important era of overall production at the Rock Creek deposit began in 1901, when articles of incorporation were filed for the American Gem Mining Syndicate, based in St. Louis. The company was incorporated by David Jankower of London and New York; Paul A. Fusz, Moses Rumsey, and Charles D. McLure of St. Louis; L.S. McLure of San Diego; and William Knuth of Helena. The American Gem Mining Syndicate was capitalized with $300,000, with Jankower holding 299,996 shares and one share each distributed to Fusz, Rumsey, A.B. Ewing, and C. McLure (“Sapphire mined on Rock Creek after 1892 discovery there,” 2019). In 1902, the American Gem Mining Syndicate patented claims at the Ruby Placer in upper Sapphire Gulch, the Star Placer in lower Sapphire Gulch, and the Anaconda Placer in Anaconda Gulch. The company would continue acquiring land and patenting placer claims through 1915 (figure 10).
As with the Missouri River deposit, water has always been the limiting factor in mining operations at Rock Creek. Water flowing through the gulches was insufficient to sustain major mining activity. With the vast capital it raised, the American Gem Mining Syndicate solved this problem at Rock Creek by constructing a series of ditches and flumes. The first was Cralle’s Ditch, likely in 1904, which brought water from Stony Lake to the McLure placer at the upper end of Sapphire Gulch, although the water was likely used in Anaconda Gulch as well. In 1912, the Upper Sapphire Ditch was completed, taking water from the North Fork of the West Fork of Rock Creek to the head of North Fork Coal Gulch and the head of Anaconda Gulch. An additional unnamed ditch was also constructed, bringing water from the West Fork of Rock Creek upstream 1,200 m to the west to Sapphire Gulch.
The water from these ditches and flumes supported mining activities during the two most productive years of 1906 and 1907, which yielded 6,482.3 kg and 6,639.9 kg of sapphire, respectively. Mining was carried out by either ground sluicing or hydraulic mining. During these years, mining activities were intense and the mine often operated 24 hours a day. From 1903 to 1928, while the American Gem Mining Syndicate was working the deposit, 46.4 metric tons of sapphire were produced (Berg, 2014).
Meanwhile, times were changing with increasing competition from synthetic ruby and sapphire, especially starting in the 1920s. Although Auguste Verneuil grew his first flame-fusion rubies in 1883, the material did not become widely available until the 1910s, when he expanded his enterprise. As more and more flame-fusion ruby and sapphire reached the market, and as the quality of the laboratory-grown material improved, Montana sapphire could not compete. Facing a dire financial situation, the American Gem Mining Syndicate sold most of its claims to Charles Carpp Jr. and J. Walt Kaiser. The two worked the deposit until 1943 and produced about 2.8 metric tons of sapphire, largely through hydraulic mining (Clabaugh, 1952). They apparently intended to sell the sapphires as gemstones but were unsuccessful due to the typically pale colors of the natural rough. Therefore, most of the production still went to the industrial sapphire market.
After Carpp and Kaiser stopped mining in 1943, the Rock Creek properties passed through several hands. Carpp and Kaiser sold to Sally and Bill Eaton, who operated a fee dig for tourists. The Eatons in 1966 sold the Meadow property along the West Fork of Rock Creek to Marc Bielenberg and the gulch properties to Wilfred Chaussee, who formed the Chaussee Sapphire Corporation and continued running the tourist operation. This operation was later passed to Wilfred’s daughter and son-in-law, Yvette and Kenneth Clevish. The Clevishes sold in 1980 for $1 million to Ted Smith, who renamed the operation the Gem Mountain Sapphire Corporation. In the meantime, Marc Bielenberg sold the Meadow property in 1978 to Skalkaho Grazing, Inc., which constructed a floating wash plant in 1982 near the confluence of the Anaconda and Coal gulches with the West Fork of Rock Creek.
Still, mining activities during this period never approached the production by the American Gem Mining Syndicate in the early twentieth century. However, another technological advance on the horizon would change the fate of Rock Creek sapphire. In the 1970s, the Thai gem trade developed and perfected high-temperature heat treatment procedures to dramatically improve the color and clarity of pale and milky sapphires, creating deep, rich blue sapphires. The original material used was the milky geuda sapphire from Sri Lanka, but it was soon realized that the same heat treatment method could be applied to secondary Montana sapphire with outstanding results. In the early 1980s, a Mr. McCarthy of Helena was reportedly the first person in Montana to use this high-temperature heat treatment to improve the color of a Montana sapphire; however, the process was perfected in a technical sense in the 1990s and scrupulously detailed in the work of Emmett and Douthit (1993).
With the ability to transform color through heat treatment, the economics of mining and marketing Montana sapphire changed for the better. In 1994, a new player entered the scene when American Gem Corporation purchased much of the gem-producing areas of the Rock Creek district, including the Dann Placer and Anaconda Bench as well as the holdings of the Gem Mountain Sapphire Corporation. All told, American Gem Corporation reported production of more than four million carats of gem-quality rough sapphire, of which more than two million heat-treated faceted sapphires to date have been sold into the international market (Kane, 2020). While American Gem Corporation, a public company listed on the Toronto Stock Exchange, carried out a high-profile marketing campaign, their efforts never materialized into a steady supply of gems, and the company reorganized as an e-commerce entity in 1999. At this point, Chris Cooney purchased their claims at the Anaconda and North Fork Coal gulches, where he now operates a mining and fee-dig tourism operation called Gem Mountain. At the same time, logging company RY Timber acquired the rest of the patented claims in the Rock Creek deposit.
The next chapter in the Rock Creek story is being written by Potentate Mining. In 2011, Potentate purchased Eureka Gulch on the north side of the mountain and became the largest landholder on Gem Mountain in 2014 after purchasing most of the south side of the mountain from RY Timber. Over the years, Potentate has actively developed its claims and now operates a modern operation utilizing state-of-the-art mining technologies to produce sapphire in an environmentally friendly manner while making efficient use of limited water resources. Among the advances implemented are a new gravel-processing facility with a separate gold circuit to maximize gold recovery, as well as a water clarifier to ensure the return of clean water to the pristine mountain streams. In 2020, Potentate finalized the purchase of the Meadow property and now controls the bulk of the sapphire-bearing areas of the Rock Creek deposit. Potentate is pursuing an aggressive marketing campaign to put Montana sapphire in a prominent place in the world market.
Dry Cottonwood Creek. Sapphires were first found by gold prospectors in the South Fork of Dry Cottonwood Creek near the city of Butte in 1889 (Kunz, 1894). Mining commenced shortly thereafter by the Northwest Sapphire Company of Butte, which employed hydraulic mining as well as dredging operations at the South Fork of Dry Cottonwood Creek (Struthers and Fisher, 1903). One of the more significant mining enterprises involved the Variegated Sapphire Company, which in 1905 installed a bucket-line dredge at the Grand Pre Flats, an open area along the South Fork of Dry Cottonwood Creek. The dredge worked an area 24–30 m wide, removing 12 m of gravel per day and recovering both gold and sapphire. In 1907, ownership of the enterprise transferred to Mr. West Dodd of Des Moines, but this operation ended by 1911, only to be briefly revived in 1914 by Nat Simon. However, Simon abandoned it after one season of low gold yield and sapphires that were pale and not marketable. In 1908, the Consolidated Gold and Sapphire Mining Company of Butte installed another dredging operation about 1.5 km from the Grand Pre Flats, downstream from the South Fork of Dry Cottonwood Creek. This effort was short-lived as well due to the low concentration of gold and the difficulty of selling the sapphires in the gem and jewelry industry.
Interest in the Dry Cottonwood Creek deposit revived during World War II due to the shortage of industrial laboratory-grown sapphire coming from Europe, but there was no production in those years. Significant mining did not resume until the 1990s, when American Gem Corporation acquired claims here and at Rock Creek and Missouri River. American Gem Corporation purchased land in 1993 from Scott Wurster, who had in turn acquired it from Marc Bielenberg, who had previously owned claims in the Rock Creek district. The advent of high-temperature heat treatment made Montana sapphire much more attractive to the gem and jewelry industry with the rich vivid blue and fancy-color gems that could be produced. In 1995, American Gem Corporation mined 400,000 carats of sapphire from Dry Cottonwood Creek (Kane, 2020). However, the Dry Cottonwood Creek sapphires did not react as well to heat treatment as those from Rock Creek (J.L. Emmett, pers. comm., 2022), and American Gem Corporation abandoned operations there in 1996 to focus on Rock Creek. In the same year, Gem River Corporation began mining sapphires in the Grand Pre Flats, but mining stopped in 1998 and the area was reclaimed for nature. No serious mining efforts have taken place at Dry Cottonwood Creek since then. However, the Dry Cottonwood Creek sapphires do react well to heat treatment in an oxidizing environment to produce variegated yellow and orange colors (R.E. Kane, pers. comm., 2022). With changing market behavior and growing interest in such fancy-color sapphires, Dry Cottonwood Creek has the potential to become a viable mining property.
CHARACTERISTICS OF THE DEPOSITS
Geology. The rugged terrain and landscapes of western Montana are the product of both ancient and modern geological forces at work. The very oldest rocks encountered in the sapphire-producing areas belong to the Proterozoic Belt supergroup, which forms the bedrock underlying much of the Missouri River and Rock Creek deposits and also represents much of the Belt Mountains seen to the east of the Missouri River deposits. The Belt supergroup includes metasedimentary rocks deposited between 1470 to 1370 Ma in a sedimentary basin formed at the margin of the North American Craton and some other unknown ancient landmass. The Belt supergroup, an accumulation of sediments an astonishing 15 km thick at its maximum extent, provides many clues to the ancient movement of the earth’s protocontinents. But these primeval geological formations serve as nothing more than the backdrop of the gemological story. At the Missouri River, these ancient formations are simply the bedrock upon which the sapphire-bearing gravels were deposited. The Proterozoic Belt supergroup formation here is in the form of an argillite, a low-grade metamorphosed version of a sedimentary shale or claystone. At Rock Creek, the Belt supergroup rocks include metasedimentary rocks that underlie Cenozoic volcanic formations, which are apparently related to the gem deposits.
The story of sapphire formation begins much later in the earth’s history, during the Cenozoic era. While the sapphires occur in secondary deposits, field observations indicate in each case that the original host rock is most likely related to Cenozoic volcanic formations erupted around 50 Ma. From about 160 to 50 Ma, the entire western seaboard of North America was a convergent margin, with the ancient Farallon plate being subducted beneath the North American continent. Subduction of the Farallon plate led to significant volcanism throughout the western United States, Canada, and Mexico. The volcanic formations in the region of the sapphire deposits are related to the late stages of this period, when a flat-lying Farallon slab started to roll back and eventually detach as it continued its descent into the earth’s mantle. As the sapphire-bearing volcanic formations were weathered and broken down, the sapphires were liberated and entrained in the rich gem gravels being mined to this day. The following sections will briefly describe the relevant formations in each deposit.
Missouri River Geology. There is very little geological context remaining to explain the deposits at Missouri River, where sapphires are recovered from gravel beds sitting atop bedrock terraces above water level (figure 11). The sapphire-bearing gravel beds are composed predominantly of locally sourced material. This means there are limited options to consider for potential bedrock sources of the sapphire. Further complicating matters is that the Missouri River here has been flooded by the construction of the Hauser Dam and subsequent creation of Hauser Lake, limiting potential exposures of sapphire-bearing lithologies. The Proterozoic Belt supergroup argilites immediately underlying the gravel beds are not suitable lithologies for corundum formation, and the same is true for the Paleozoic and Proterozoic metasedimentary rocks of the nearby Big Belt Mountains and the Paleozoic sedimentary rocks in the Spokane Hills.
What little evidence we have comes from one small sapphire-bearing sill found at French Bar.1 Based on chemical analysis and petrographic observations of thin sections of the volcanic rock, Berg and Palke (2016) described it as a basaltic trachyandesite composed of biotite and augite phenocrysts in a matrix of plagioclase microlites. Rare corundum-bearing xenoliths have been found composed of calcic plagioclase, augite, garnet, margarite, and spinel (Berg and Dahy, 2002).
1Note that while sapphire-bearing sills have been reported at both French Bar and Ruby Bar, Berg and Landry (2018) pointed out that historical analysis proved these two bars are the same, and thus it is likely that only one sapphire-bearing sill has been discovered.
Importantly, Berg and Palke (2016) used LA-ICP-MS to analyze the chemistry of sapphires found in situ in this (sub)volcanic formation, and the results demonstrated that their trace element profiles are consistent with the gem sapphires found in the gravel beds. This indicates that these volcanic formations are viable sources of the Missouri River sapphires. While this single volcanic formation is surely too small and has too low a sapphire concentration to have supplied gems for the entire Missouri River deposit, it suggests a likely geological origin of the sapphires, with the implication that other similar sapphire-bearing (sub)volcanic formations must have been present but were weathered away almost entirely. Additionally, many sapphires from the Missouri River are found with thin encrustations of spinel adhering to their surface. This spinel is inferred to result from a reaction between the corundum and magnesium and iron sourced from mafic magmas that transported the sapphires to the surface. This spinel rim is not found on sapphires from Rock Creek or Dry Cottonwood Creek and is not even found on all Missouri River sapphires.
Rock Creek Geology. In the Rock Creek area, sapphires are found in gravel beds in gulches cutting into Gem Mountain (figure 12). The dominant formations in the area are rhyolitic lava flows, dikes, and tuffaceous and volcaniclastic formations that overlie metasedimentary rocks of the Proterozoic Belt supergroup. Given that sapphires are found in the gulches cutting up into the mountain, their source must have been local. The volcanic formations here are relatively silica-rich and rhyolitic in composition. Rhyolite lava flows cap the ridges separating the sapphire-rich gulches, while the more readily eroded tuffs and volcaniclastic units are less well preserved. No other volcanic formations have been identified in the immediate area, although it has been postulated that there may be hidden plugs of lamprophyres or other mafic volcanics in the area that have supplied the sapphires (Barron and Boyd, 2015). Notably, Berg (2014) did identify a basaltic dike exposed along a road cut 4 km east of Sapphire Gulch. No sapphires have been found in this dike, but to the authors’ knowledge it has never been studied thoroughly. Still, this basaltic dike is likely too small and too far from the deposit to be a viable source of the sapphires. While no sapphires have been found in situ in any volcanic rocks here, Berg (2014) did identify some with what was described as a “rhyolitic” encrustation. This encrustation was observed on another sapphire by Belley (2022), with scanning electron microscopy (SEM) images confirming that the adhering crust was composed of quartz, plagioclase, and potassium feldspar. Importantly, Rock Creek sapphires are never found with the spinel encrustation found on some Missouri River sapphires, which is likely formed by interaction with magnesium and iron in the mafic transporting magma at Missouri River. This observation seems to suggest a mafic source is less likely at Rock Creek. With the enormous volume of sapphire produced at Rock Creek, the original source rock must have been extensive, decreasing the likelihood of a hidden source that was weathered away. Given the prevalence of rhyolitic volcanics in the area, the evidence suggests that rhyolitic volcanism is a likely source of the sapphires in this deposit.
Dry Cottonwood Creek Geology. Sapphires at Dry Cottonwood Creek are found in gravels and secondary deposits. Bedrock is composed of volcanic formations from the Eocene-age Lowland Creek Volcanics. These overlie Cretaceous granitic rocks of the Boulder Batholith, which contacts the Lowland Creek Volcanics near the western boundary of the Grand Pre Flats on the South Fork of Dry Cottonwood Creek. The Lowland Creek Volcanics are predominantly composed of welded tuffs and lava flows of dacitic composition. No other volcanics or other geological formations exist in the immediate area. While no thorough field studies have been performed to identify the source of the sapphires, the Lowland Creek Volcanics seem to be a likely original bedrock source, given the likely volcanic origin of all Montana sapphire and the paucity of other possible sources.
Origin of the Secondary Montana Sapphires. Given the relative lack of hard rock geological evidence in these secondary deposits, one must search elsewhere for clues about the ultimate origin of these sapphires. In fact, while the original sapphire-bearing host rocks have mostly weathered away, remnants of the ancient sapphire-producing geological environments have been preserved within the sapphires as inclusions. Besides hexagonal bands of silk and rutile particles, among the most commonly encountered inclusions are fluid inclusions of an unusual nature. These often have the appearance of typical two-phase fluid inclusions seen in other gems. However, if one were to cut into one of these fluid inclusions, the fluid material would be frozen in place and not leak out of the sapphire as expected. These fluid inclusions represent blebs of magma and indicate that the fluid present during sapphire growth was a molten silicate rock. Upon transport to the surface in volcanic events, the sapphires must have cooled quickly enough to quench these melts into glasses.
The compositions of the melt inclusions are broadly similar across the three deposits, suggesting similar conditions of geological formation. The melts coexisting during sapphire growth were poor in MgO and FeO, moderately enriched in Na2O, K2O, and CaO, and (surprisingly) relatively rich in SiO2. The melts can be classified as dacites or rhyolites according to the total alkali silica (TAS) diagram of Le Bas et al. (1986). Of particular note is that these melts are all quartz-normative, meaning that if cooled in an isolated system, they should have precipitated quartz. This seems counterintuitive at first, as it is well known that corundum and quartz cannot coexist under ordinary geological conditions. However, it is possible for corundum to coexist with a quartz-normative melt in the case of an incongruent (or peritectic) melting reaction. The presence of quartz-normative melts then suggests a genetic model in which the secondary Montana sapphires grew through partial melting of some aluminum-rich rock (e.g., an anorthosite or some similar plagioclase-rich igneous rock). In this model, the magmas that transported the sapphires to the surface in volcanic events might have also been responsible for sapphire formation by initiating partial melting of the proposed aluminum-rich protoliths. In this scenario, the sapphires could still be foreign to the transporting magmas but would not be accidental xenocrysts. In fact, based on observations of rhyolitic secondary melt inclusions, Palke et al. (2017) suggested the possibility that some sapphires at Rock Creek may not be xenocrysts at all if the partial melting event that produced the rhyolites seen at the surface also produced the sapphires entrained in this magma. Of course, proving or disproving this hypothesis would be challenging. At Missouri River, the basaltic trachyandesite magma that likely transported some of the sapphires is significantly different from the melt inclusions seen in those stones, confirming their identity as xenocrysts. The Lowland Creek Volcanics at Dry Cottonwood Creek have not been thoroughly studied, and their relation to the melt inclusions seen in those sapphires is less clear.
The sapphires’ origin from some calcium- and aluminum-rich protolith such as an anorthosite is also supported by the prevalence of calcium- and aluminum-rich mineral inclusions such as anorthite-rich feldspar and (clino)zoisite. Other genetic models have been proposed, mostly based on the use of trace element chemistry wherein the low gallium and other trace element patterns were thought to suggest a metasomatic or metamorphic origin for the sapphires (Garland, 1992; Zwaan et al., 2015). Such an origin, however, seems to be contradicted by the pervasiveness of silicate melt inclusions, which indicate that the sapphires grew in the presence of a silicate magma.
GEMOLOGICAL CHARACTERISTICS OF SECONDARY MONTANA SAPPHIRE
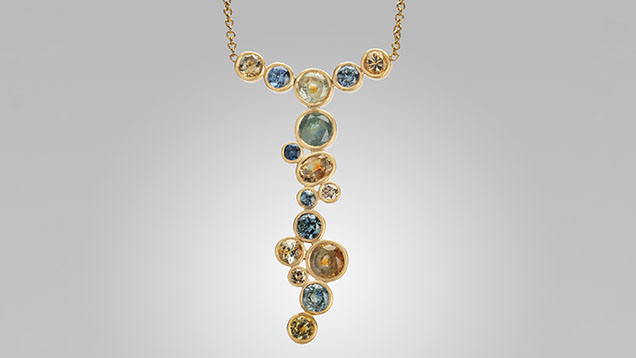
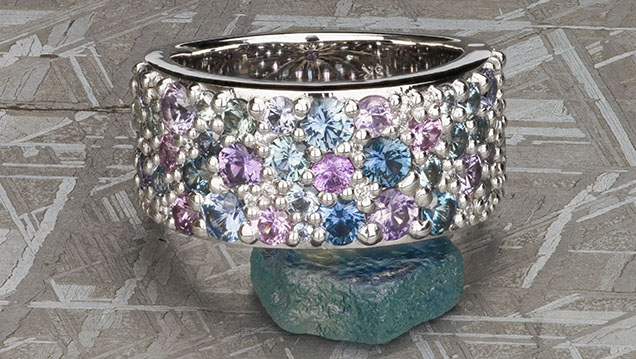
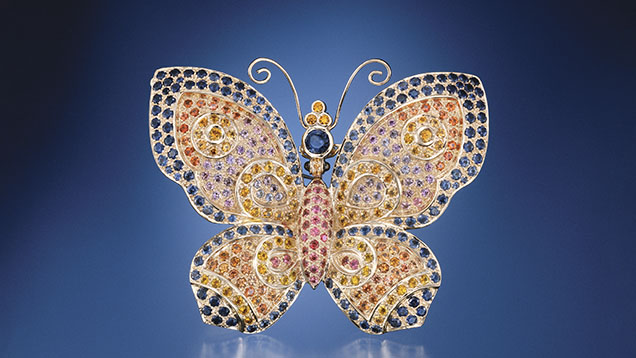
Physical Properties. Sapphires from Montana’s secondary deposits yield some unique characteristics that make them distinct from other sapphires around the world. While basic physical properties such as refractive index and specific gravity offer no significant differences, the inclusion suite and trace element chemistry can help to distinguish secondary Montana sapphire. However, it should be noted that the characteristics of individual faceted sapphires from Montana’s secondary deposits overlap significantly, and these gems cannot currently be reliably separated from each other.
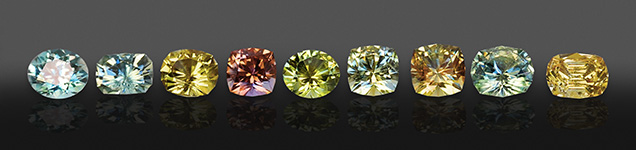
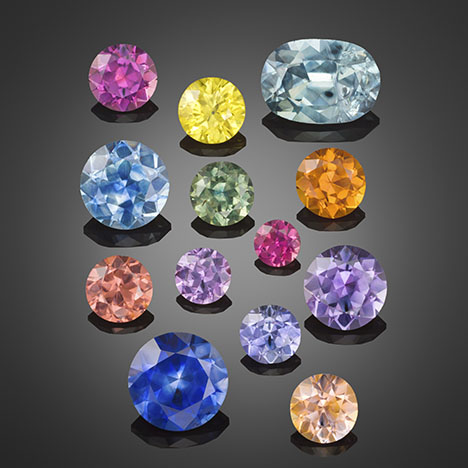
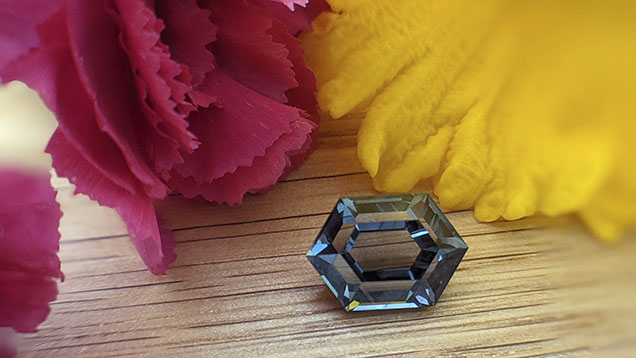
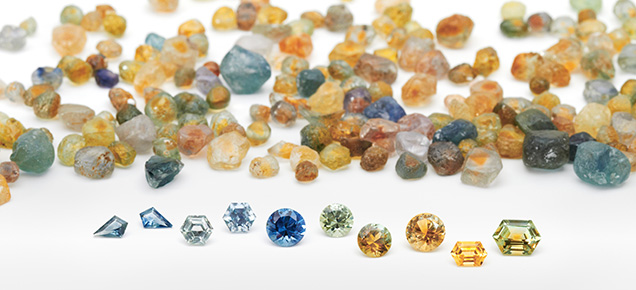
In general, sapphires from Montana’s secondary sources naturally occur in a wide range of hues, including blue, green, yellow, orange, pink, and purple (figures 13–19). Most of these colors are somewhat pale, especially when unheated (figures 13 and 14). Vibrant, saturated colors do occasionally occur naturally, but they are more common in heated stones (figures 15–17). Montana sapphire has become increasingly popular in nonstandard cutting styles (figures 18–19). Parti-colored sapphires are frequently observed, especially for heated stones with a yellow or orange core and a blue, green, pink, or violet rim (figure 20). Some sapphires exhibit a weak to moderate color change when observed in incandescent to daylight illumination (figure 21). Colors generally change from shades of pink or orange (incandescent) to green or violet (daylight). Rubies are also sometimes found (figure 22). Palke and Hapeman (2019) reported only 29 g of rough ruby crystals recovered from more than 400 kg of mine production at Potentate’s operation at Rock Creek. While truly red gem corundum from Montana’s secondary sapphire deposits are rare, examination of the collection of Robert Kane (Fine Gems International, Helena) uncovered numerous examples of rubies from color-graded parcels of pink sapphire. While rare, these Montana rubies do exist and may be encountered in the market. Other examples of Montana ruby are known, including one particularly large faceted gem weighing 1.70 ct (Malaquias, 2018).
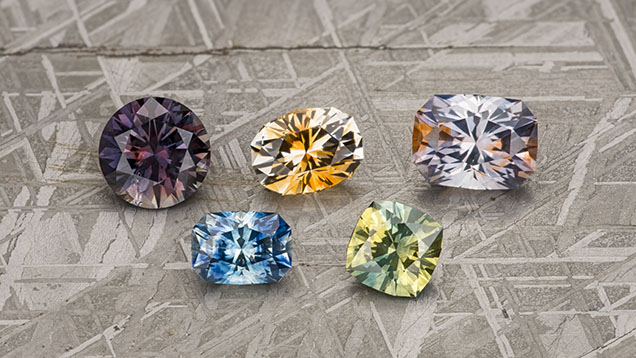
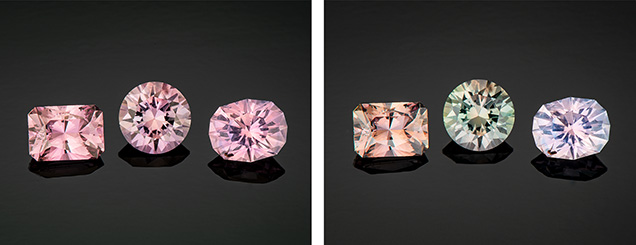
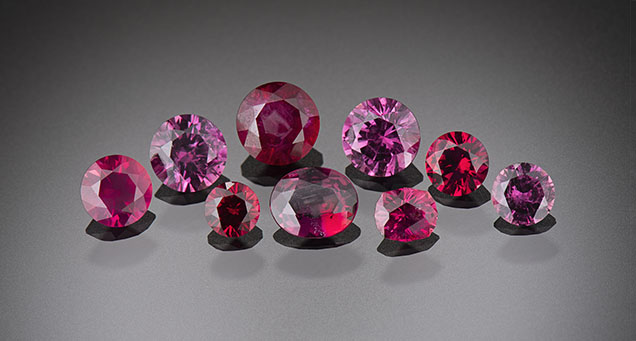
Sapphire rough from these deposits generally occurs as small crystals measuring a few millimeters in diameter, but larger stones have been found. Notably, the unheated blue sapphire known as the “Big Sky” sapphire, which sold in 2015 for $394,000, weighed 12.54 ct (Kane, 2020). Rough crystals as large as 37.78 ct have been reported from Eldorado Bar (Berg and Landry, 2018), while the Rock Creek deposit has produced stones as large as the faceted 12.62 ct heated stone recovered by Potentate Mining known as the “Montana Queen” (@potentatemining, May 27, 2019). Potentate also recovered a large crystal from Rock Creek in 2018 weighing 64.14 ct, known as the “Ponderosa” sapphire, as well as a 67.15 ct yellow sapphire recovered in 2021 that cut a 12.18 ct stone called the Yellowstone sapphire. This is currently part of the Somewhere in the Rainbow collection (again, see figure 1). Rock Creek also produced a notable 39.14 ct stone in 2008 that was recovered from the Anaconda Bench at Gem Mountain (Berg, 2014). The largest crystal reported from Dry Cottonwood Creek weighed 22 ct but had a flat shape and therefore a low yield when cut into a gem (Berg, 2007). While small stones under a carat are the main production from Rock Creek, figure 1 shows a suite of the finest and largest sapphires produced by Potentate during the 2021 mining season in their rough and polished states.
Optical Measurements and Specific Gravity. Standard gemological testing of Montana sapphire reveals properties one would expect for corundum. The average refractive index measured is 1.760–1.770, and the birefringence typically ranges from 0.008 to 0.010. The specific gravity ranges from 3.98 to 4.01, also consistent with corundum.
FTIR spectra of Montana sapphire can show a variety of features (figure 23). Commonly seen is a weak single peak at 3309 cm–1. This peak is believed to be related to hydrogen in the corundum structure associated with titanium substituting for aluminum (Moon and Phillips, 1991). Also common in secondary Montana sapphire is a peak at about 3220 cm–1. A similar peak is often seen in Yogo sapphire as well (Renfro et al., 2018), but its origin is unknown. Finally, many Montana sapphires show the collection of broad absorption bands centered around 3000 cm–1 that is often referred to as the “Punsiri peaks” but will be referred to here as the acceptor-dominated 3000 cm–1 series, given that these peaks are related to hydrogen associated with acceptor ions, in this case Mg2+, in the corundum (Fukatsu et al., 2003; Sangsawong et al., 2016). The acceptor-dominated 3000 cm–1 series is not an indicator of heat treatment for Montana sapphire, as these peaks sometimes occur naturally. In fact, this series of peaks is very common in unheated Rock Creek sapphire but only occasionally found in Dry Cottonwood Creek sapphire and almost never in Missouri River sapphire. The commercial importance of this finding will be discussed later in the section dealing with heat treatment. The peak at 3220 cm–1 can be used as an indicator of a secondary Montana or primary Yogo origin, but the other FTIR features such as the 3309 cm–1 peak and the acceptor-dominated 3000 cm–1 series of peaks are observed in sapphires from many other origins. Montana sapphire from all the secondary deposits may also show mineral inclusion peaks in their FTIR spectra, especially for kaolinite or gibbsite.
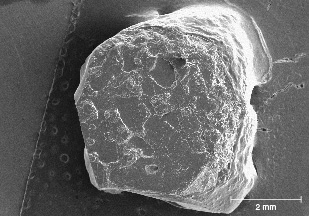
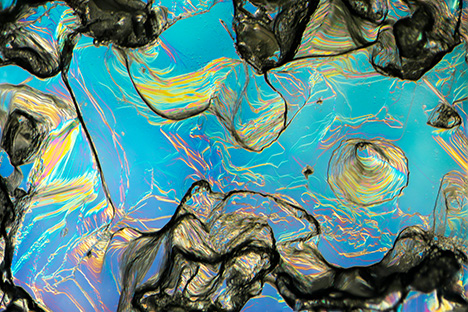
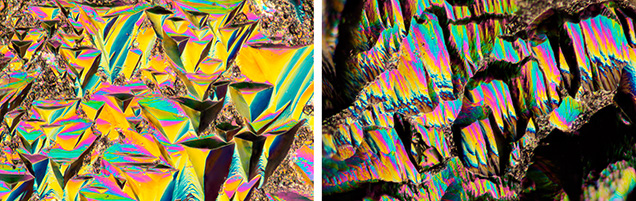
Shape of Rough Crystals/Morphology. The sapphire from Montana’s secondary deposits is generally tabular or blocky in shape and can also appear somewhat less commonly as hexagonal prisms. The rough stones also tend to show a rounded appearance, which might cause one to suspect they have been subjected to rounding by abrasion from water transport. However, close examination with a microscope often reveals pronounced geometric patterns on the surface with either the hillocky-type texture seen at Rock Creek or dissolution-type etch features (figures 24–26). In fact, the sapphire morphology from the three deposits generally differs enough to allow identification of the origin of parcels of rough sapphires, even if individual stones cannot be traced back to their source. For instance, some 25% of sapphires from Missouri River have an incomplete or spotty dark green coating of spinel in depressions on the surface, as seen in the SEM image in figure 27. This feature is not seen in sapphire from Rock Creek or Dry Cottonwood Creek. Dry Cottonwood Creek sapphire shows etching along twinning planes as seen in figure 28, which is less common in Rock Creek and Missouri River sapphire. Finally, Rock Creek sapphire often has growth hillocks on the basal surface, some with flat surfaces resembling miniature mesas (figure 24). Examples of rough from each deposit are shown in figure 29.
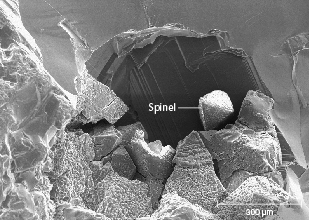
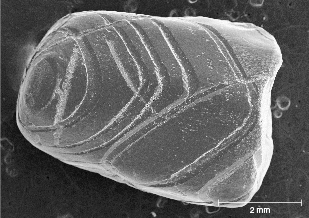
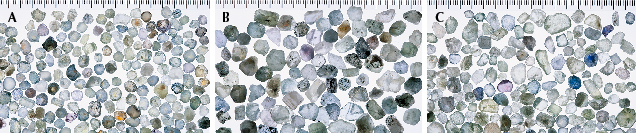
Figure 30 shows typical shapes for the crystals found at all three locations. The two most commonly encountered shapes are hexagonal prismatic crystals with slightly developed rhombohedral faces (figure 30, A and B). Many show development of the hexagonal bipyramidal faces as well (figure 30, A and C). Crystals typically exhibit six-fold or three-fold symmetry on the basal pinacoid; figure 30B shows a classic raised trigonal pyramid on the basal pinacoid. There are slight variations between the three deposits in the prevalence of the various crystal shapes. For example, the majority of the crystals from Dry Cottonwood Creek are tabular hexagonal crystals (figure 30C), while elongated hexagonal prisms (figure 30D) are particularly common at Missouri River. At Rock Creek, most of the crystals have been so heavily etched/resorbed that most of their crystal faces are obscured, although color zoning and the patterns of rutile inclusions follow these crystal faces and can reveal the structure even in heavily etched crystals. Despite this variation at each locality, crystals of all of these shapes can be found at any of the deposits.
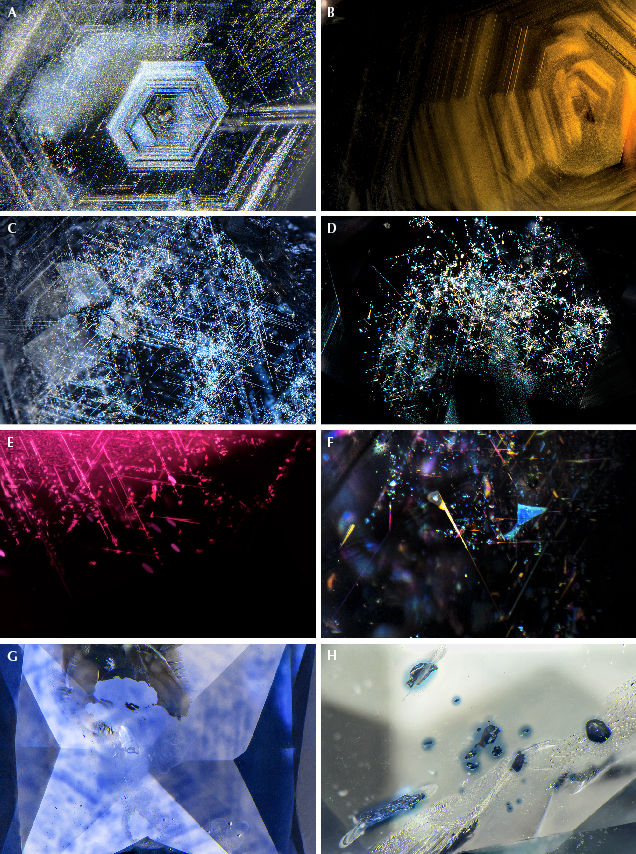
Microscopic Features. Secondary sapphires from Montana contain a wide array of inclusions, and these are a powerful tool in confirming a Montana origin. In natural, unheated Montana sapphire, the most common features are bands of rutile silk, often arranged in hexagonal patterns, either as dense hexagonal clouds (figure 31, A and B) or in a loosely packed but overall hexagonal pattern (figure 31, C and D). The individual particles making up the clouds may be relatively long, slender needles varying in size all the way to very short, stubby, or dusty particles. In some cases, the particles take on a flattened, platelet-like shape and are highly reflective, often showing iridescent interference colors with the use of an intense fiber-optic light due to a thin-film effect (figure 31, D–F).
Given that the majority of Montana sapphires on the market have been heat treated to enhance their colors, attention must be paid to the inclusions that can help the gemologist confidently identify this treatment. The most obvious effect of heat treatment on Montana sapphire is internal diffusion of blue coloration from rutile inclusions and silk. This can be seen as patchy blue color zones that precisely match the original patterns of the rutile silk. This is most easily observed using diffuse transmitted illumination, but fiber-optic illumination can be useful as well. This will lead to patchy blue color zoning surrounding bands of silk that are partially or nearly completely dissolved into the corundum lattice (figure 31G) as well as dark, inky spots surrounding protogenetic rutile inclusions (figure 31H). More examples will be shown in the section on heat treatment below.
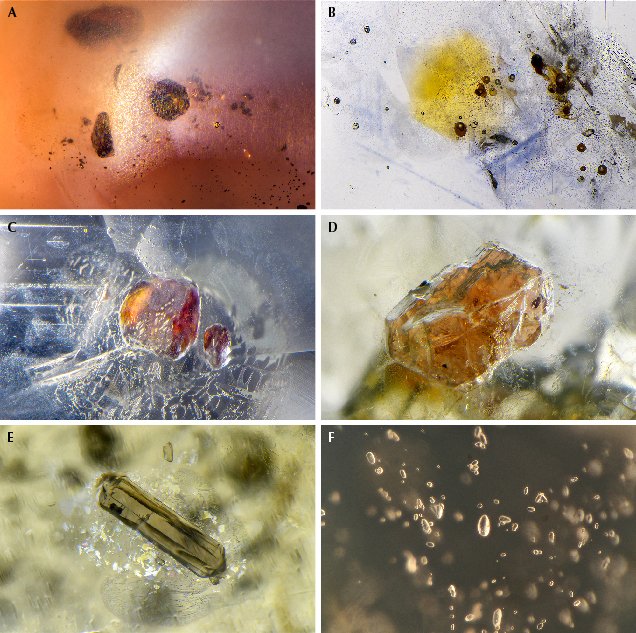
On rare occasions, rutile silk may be dense enough to produce a star sapphire when cut in cabochon form (figure 32A). Yet star sapphires from Montana are exceedingly rare, and the star is usually poorly developed. Many of them show interesting coloration, with yellow or orange zones in the core of the crystal, which is most prominent in heat-treated stones (figure 32B). Twinning with tube-like structures at the intersection of twin planes is fairly common in sapphires from Dry Cottonwood Creek but may occasionally be seen in sapphires from Rock Creek and Missouri River. These features are observed in sapphires from other deposits as well (Hughes, 2017) and have been referred to as “Rose channels” (Notari et al., 2018). (Note that Rose channels have been referred to erroneously in the past as “boehmite needles.”) Large, blocky protogenetic rutile inclusions are common in stones from all the Montana secondary deposits (figure 32C). In this study, garnets were occasionally encountered in sapphires from Dry Cottonwood Creek (figure 32D). Although Zwaan et al. (2015) observed garnet inclusions in sapphire from Rock Creek and Missouri River, the present authors have not; these inclusions are likely rare compared to material from Dry Cottonwood Creek. Garnet inclusions could then be considered a likely indicator of Dry Cottonwood Creek origin. Sapphires from all three deposits are likely to contain inclusions of clinozoisite, which takes on an elongate form (figure 32E), sometimes with multiple fractures or cleavages perpendicular to the direction of elongation. Sapphires from Dry Cottonwood Creek are also more likely to contain zircon inclusions, especially in clusters and galaxies of crystals resembling rice grains (figure 32F). Zircon inclusions are rare in sapphire from Rock Creek and Missouri River.
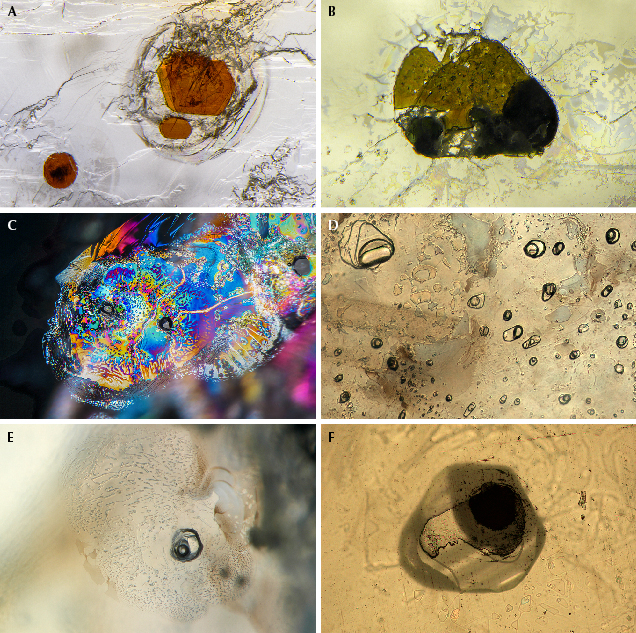
Mica inclusions may be seen and range from nearly colorless to pale shades of yellow to occasional dark orange hues (figure 33A). Colorless rounded feldspar inclusions are fairly common as well from all three deposits. Note that a number of other mineral inclusions were documented by Zwaan et al. (2015) and compiled in a table of occurrence. While many of the sapphires they studied appear to have been heated, Zwaan et al. (2015) report a more extensive list of minerals identified in their study and from other literature sources as well.
In some rare sapphires from Missouri River, unusual multiphase inclusions have a negative crystal morphology in which the shape of the inclusion is constrained by the crystal forms of the corundum host and not by the minerals inside the inclusion. These multiphase inclusions are composed of mica, spinel, and some other unidentified phases (figure 33B). These inclusions are interpreted to have been entrapped initially as a melt phase, which recrystallized post-entrapment. In fact, melt inclusions with a negative crystal shape are quite common in secondary Montana sapphire. They often are surrounded by decrepitation halos, which may be partially healed and show iridescent interference colors (figure 33C). In many cases, these inclusions look like standard two-phase fluid inclusions (figure 33, D and E). However, when one of these inclusions is cut into at the surface of a stone, the apparently fluid phase does not flow away but is frozen in place (figure 33F). These inclusions were trapped as a relatively silica-rich melt (Palke et al., 2017) that coexisted with the sapphires as they were growing. After being emplaced at the earth’s surface, the sapphires must have cooled quickly enough to quench the melt phase into a glass. Note that while these inclusions resemble the “melted crystal” inclusions seen in heat-treated sapphires, it is not correct to apply this term to these inclusions. These are properly called “melt inclusions,” as they were included as a melt and do not represent crystalline inclusions melted by artificial heat treatment. Therefore, observation of this type of inclusion is not, in and of itself, evidence of heat treatment in Montana sapphire. Identification of heat treatment is more reliably carried out by observation of the altered crystalline inclusions, the altered nature of rutile silk particles, and/or color diffusion around rutile or silk particles.
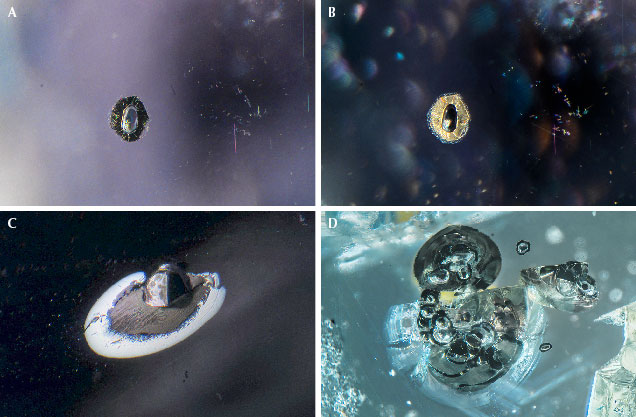
Also worth mentioning are sulfides assuming a negative crystal shape. These inclusions are dark black in darkfield illumination but may display a highly lustrous surface with the use of a fiber-optic light (figure 34). They are always accompanied by a decrepitation halo where sulfide material has leaked out into the surrounding corundum host. The negative crystal morphology and observation of a polyphase mineral assemblage by SEM analysis suggests that these are an additional type of melt inclusion. In this case, a sulfide melt must have been present during sapphire formation in addition to the more silica-rich melts described above. In the authors’ experience, these sulfide melt inclusions are seen almost exclusively in sapphires from Missouri River and Dry Cottonwood Creek, though they are not common even then. Although sulfide inclusions have been noted in a few Rock Creek sapphires (J.I. Koivula, pers. comm., 2022), screening of thousands of samples by the authors has not identified this inclusion in Rock Creek sapphire.
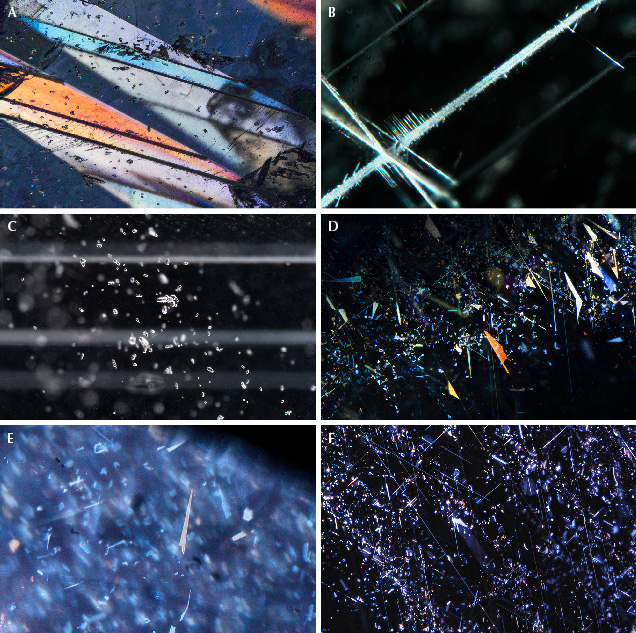
Inclusions/Microscopic Observations of Other Non-Classical Sapphires. The sapphires most likely to be confused with Montana sapphire are those from Songea in Tanzania. Sapphires from Umba in Tanzania may also show some generally similar characteristics, but the inclusions are generally distinct enough to clearly separate them from secondary Montana sapphires. The first thing one might notice is the overall similar color range among all these deposits, which tends to include light-toned, low- to medium-saturation pastel hues of blues, greens, yellows, oranges, purples, and pinks. Beyond the initial impression from the bodycolor, one may find evidence to separate these sapphires down the ocular barrel of a microscope. Umba sapphires especially tend to have distinct inclusion features, the most common of which is heavy, repeated twinning. Under cross-polarized light, the variously twinned sectors may create a unique mosaic of colors (figure 35A). The boundaries between twinned sectors are often prominent and may be filled in with some secondary aluminum oxy-hydroxide minerals (figure 35B). Fields of clustered and single zircon inclusions are quite common in Umba sapphires (figure 35C). Dense aggregations of silk are not as common as in Montana sapphires; however, the silk that can be seen often takes on a platy but very angular appearance (figure 35, D–F).
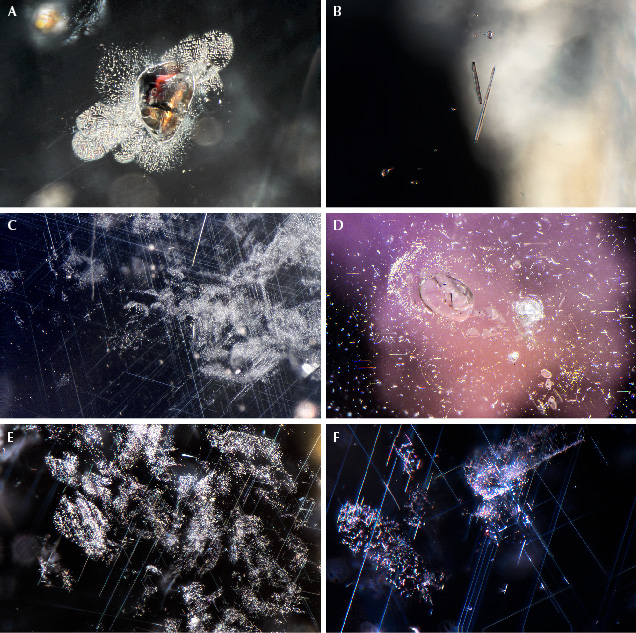
Songea sapphires may have inclusion features that could look somewhat similar to secondary Montana sapphire inclusion features. Often seen are blocky to angular or needle-like protogenetic rutile inclusions (figure 36, A and B). The silk in some Songea sapphire can somewhat resemble the silk in Montana sapphire. However, it is generally much more sparsely distributed and may appear in somewhat randomly placed patches and as flecks of silk (figure 36, C–F). Songea sapphire generally does not have the well-formed hexagonal bands of silk seen in the Montana sapphire.
Trace Element Chemistry. Sapphires from the secondary Montana deposits are classified by gemologists as “non-classical” sapphires. In essence, this means they are distinct from both the typical metamorphic sapphires (from Myanmar, Sri Lanka, Madagascar, and Kashmir) and the typical magmatic, basalt-related sapphires (from Australia, Thailand, Cambodia, Nigeria, and Ethiopia). Their inability to fit into the standard metamorphic/magmatic dichotomy is also seen in their trace element chemistry. As is typical for non-classical sapphires, the secondary Montana sapphires have relatively high iron contents, suggesting a magmatic origin, while the relatively low-gallium, higher-magnesium contents and low-gallium/magnesium ratios suggests a metamorphic origin. This discrepancy was pointed out in Palke et al. (2017), which showed that the Montana sapphires plot in both the metamorphic and magmatic domains in the Fe vs. Ga/Mg and Fe-Mg×100-Ti×10 discriminant diagrams of Peucat et al. (2007) as well as the Cr/Ga vs. Fe/Ti diagram of Sutherland et al. (1998).
The general trace element characteristics of the secondary Montana sapphire deposits are summarized in table 1, where they are broadly compared with sapphires from other deposits. Note that the trace element chemistry of Montana’s three secondary deposits broadly overlaps except for a few very high-titanium Rock Creek sapphires, so they will be considered together here as a single group. There is some degree of overlap between the Montana sapphires and a small subset of higher-iron sapphires from the classical metamorphic deposits of Madagascar and Myanmar (figure 37); however, sapphires from these deposits can generally be distinguished by careful observation of their inclusion characteristics (Palke et al., 2019). The sapphires that are more likely to be confused with the secondary Montana sapphires are those from Umba and Songea in Tanzania. There is broad overlap in most trace elements for these deposits (figure 38). Iron contents are higher on average for Songea, and gallium contents of some Umba sapphires are much higher, but overall these elements cannot consistently be used for separating the Montana sapphires. But for Montana sapphires occurring in colorless, green, blue, and yellow ranges, the trace element vanadium is a useful identifier. In particular, the vanadium vs. iron plot can separate most Montana sapphires from Umba and Songea sapphire (figure 39). The caveat is that this does not apply to pink sapphire and ruby from Montana, which have higher vanadium concentrations that overlap with sapphire from Songea and Umba.
Heat Treatment of Montana Sapphires. The previous section outlined various internal characteristics with an eye toward establishing the provenance of unheated Montana sapphire from the Rock Creek, Missouri River, and Dry Cottonwood Creek deposits. However, the majority of these sapphires entering the market have been heat treated at high temperatures to improve their color, making them more desirable for use in jewelry. This treatment fundamentally alters the sapphires’ internal characteristics as well as their spectroscopic properties.
It is important to note that, due to their unique chemistry, Montana sapphires require specialized and carefully controlled heating environments that can typically be obtained only in modern resistance-style furnaces. The specific technical requirements for the heat treatment of Montana sapphire were realized in the pioneering scientific work of Emmett and Douthit (1993). These involve careful control of not only heating temperatures and duration but also oxidation environments using gas-mixing furnaces. Emmett and Douthit’s technology was used to heat the production of the American Gem Corporation in the 1990s and is currently employed by a small handful of heat treatment facilities in the United States.
This section chronicles the journey of several sapphire wafers through the heat treatment process with careful documentation of their inclusions and spectroscopy before and after heating. Additionally, the samples were cut as oriented wafers with the c-axis (or the optic axis) perpendicular to the plane of the wafer. This allows for only the o-ray spectrum to be collected, while the extraordinary ray (e-ray) spectrum cannot be observed. Note that this section is not meant to be an exhaustive review of the heat treatment process for Montana sapphires, but is intended to give readers an overview of key features that can identify heat-treated Montana sapphires and to demonstrate the effects of heat treatment on stones that are commercially available. More technical details about the heat treatment process can be found in Emmett and Douthit (1993). The first three examples were heated by Dale Siegford of the Sapphire Gallery in Philipsburg, Montana, and conditions of heating were not disclosed. The fourth and fifth examples were heated at GIA’s experimental heat treatment facility with carefully controlled conditions described below.
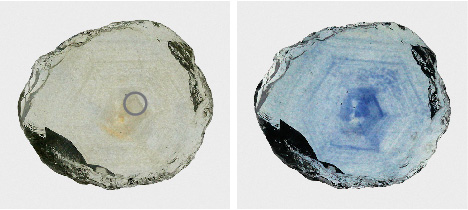
Example 1: Colorless and Cloudy to Blue. The first example illustrates the most advantageous outcome for heat treatment of Montana sapphires. The stone was initially colorless but quite cloudy and included (figure 40, left). The transmitted light photo of the unheated stone shows dense, hexagonal bands of rutile silk that scatter light, creating brown to yellowish patches. While some unheated Montana sapphire can be cut into exceptional colorless or slightly grayish gems, this stone’s cloudiness makes it undesirable for faceting. However, heat treatment causes a dramatic change in the sapphire’s appearance, creating a rich blue coloration where there were once patches of cloudy silk (figure 40, right).
The change in color is also seen in the UV-Vis spectra of this sapphire before and after heating (figure 41). The unheated sapphire showed narrow absorption bands at 377, 388, and 450 nm related to Fe3+ and a gradual upward slope from the near-infrared to the ultraviolet region, caused by scattering of light from the rutile silk. The most obvious change caused by heating is the creation of broad, intense absorption bands at 580 and 880 nm. The 580 nm band is responsible for the stone’s blue coloration, as it absorbs most of the red light passing through by way of the excitation of an intervalence charge transfer (IVCT) between Fe2+ and Ti4+ (Dubinsky et al., 2020). This band increases in intensity as rutile silk is dissolved into the corundum structure, where the titanium ions introduced can pair locally with iron ions in a charge-balancing substitution of Fe2++Ti4+ for two ions of Al3+. The origin of the 880 nm band is not well understood but may be related to clusters of Fe2+, Fe3+, and/or Ti4+ cations in the corundum structure (Hughes et al., 2017). Of particular note is that while unheated blue Montana sapphires have a UV-Vis spectrum reminiscent of a typical metamorphic blue sapphire, with an 880 nm band that is less intense than the 580 nm band (Palke et al., 2019), this heated blue Montana sapphire has a UV-Vis spectrum that resembles a magmatic, basalt-related blue sapphire, with an 880 nm band that is more intense than the 580 nm band. This “reversal” of the UV-Vis spectrum in heated sapphire has been documented by both Emmett and Douthit (1993) and Hughes and Perkins (2019), and it should provide the basis for careful application of this classification scheme for heated blue sapphires. The other notable difference for the heated sapphire is the decrease in absorption in the near-UV and blue/green region of the spectrum from about 350 to 500 nm. This is caused by the dissolution of rutile particles into the corundum structure and the consequent reduction in light scattering, which enhances the blue coloration caused by the Fe2+/Ti4+ IVCT.
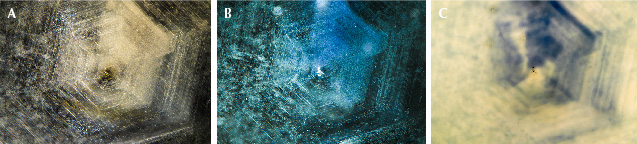
Finally, evidence of heat treatment can be found through careful microscopic observation. The pristine, unaltered silk in the unheated stone is a mixture of long needles as well as clouds of smaller particles either aligned with the hexagonal growth patterns of the corundum or forming less-regular patterns not ostensibly constrained by the corundum lattice (figure 42A). Also seen are some reflective platelet inclusions at the top left and bottom center of figure 42A. The heated stone still shows significant scattering of light from these rutile particles using fiber-optic illumination, indicating that the particles have not fully dissolved into the corundum structure. The long rutile needles have been completely destroyed by heat treatment, but the overall pattern and distribution of smaller, cloudy rutile particles has not changed dramatically (figure 42B). More conclusive evidence of heat treatment comes from observing the distribution of blue coloration seen using diffuse transmitted illumination (figure 42C) and comparing it to the distribution of incompletely dissolved rutile inclusions (figure 42B). The blue coloration in this sapphire shows a nearly exact correlation with the incompletely dissolved rutile clouds, providing conclusive evidence of high-temperature heat treatment. Also notable is that with the use of intense fiber-optic illumination, the blue coloration does seem to emanate from the especially dense cloudy regions such as the sector seen in the top center of the photomicrograph in figure 42B.
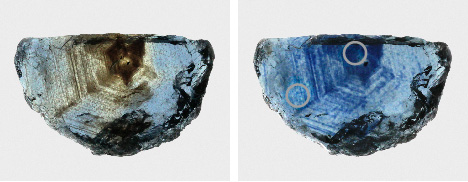
Example 2: Brownish Blue to Blue. Our second example involves a sapphire with a fairly well-developed blue coloration in its unheated state. However, the extremely dense, cloudy nature of this stone, especially in its core, would have made it unsuitable for faceting. In this stone, the silk lends a brown coloration. As shown in the before/after photos taken with diffuse transmitted light (figure 43), these brownish cloudy regions take on an intensely saturated blue color after heat treatment.
This intensification of blue color can also be seen in the UV-Vis spectra collected in two spots in the core and rim of the sample before and after heating. In the unheated state, both the core and rim have a noticeable broad absorption band at 580 nm related to Fe2+/Ti4+ IVCT as well as narrow Fe3+-related absorption bands at 377, 388, and 450 nm (figure 44). The unheated core also has a pronounced rise in absorption, and its decrease in wavelength from the near-IR to the near-UV is caused by scattering from the densely concentrated rutile silk particles. After heating, the 580 nm band increases dramatically in the core and slightly in the rim. Increased clarity (and decreased scattering) is also important for improving the blue color in the core, as there is also a significant decrease in absorption in the ~350–500 nm region caused by dissolution of light-scattering silk. Similar to example 1 above, the heated stone has what would appear to be a magmatic, basalt-related blue sapphire absorption spectrum, with the 880 nm band more intense than the 580 nm band. This is in contrast to the ostensibly metamorphic blue sapphire spectrum in the unheated stone.
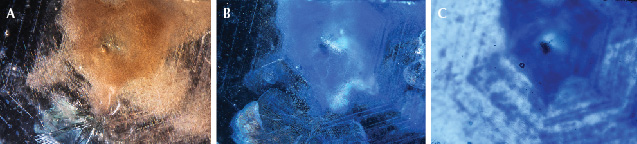
Finally, microscopic observations are useful for identifying the high-temperature heat treatment of this sapphire. The unheated sapphire shows an extremely dense concentration of silk, largely composed of medium to long needles, especially in the core (figure 45A). The long needles have become partially dissolved into the corundum in the heated sapphire (figure 45B). The use of fiber-optic illumination reveals that the silk has not completely dissolved and can still scatter light quite intensely. Importantly, in the heated stone, the light scattering off the remnant clouds has a distinct blue color as a result of the leaching of Ti4+ ions locally into the corundum. The correlation between dense blue coloration and the remnant rutile clouds can be seen more clearly with the use of diffuse transmitted illumination (figure 45C), clearly demonstrating heat treatment.
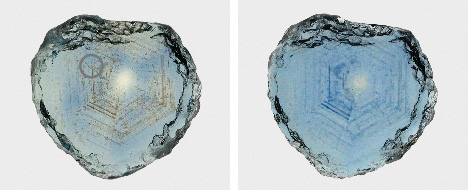
Example 3: Light Blue to Deeper Blue. The next example involves a light blue sapphire with sparse silk arranged in a hexagonal pattern, which is made obvious in diffuse transmitted light by the brownish coloration of the silk. Heat treatment increased the blue coloration by dissolving some of this silk into the corundum structure (figure 46). The change in color is, perhaps, less obvious from the UV-Vis spectra (figure 47). The absolute intensity of absorption at 580 nm is not significantly different before and after treatment. The main difference is the decreased absorption between ~350–500 nm caused by reduction in scattering when the fine rutile silk is dissolved into the corundum, allowing more blue light to be transmitted. While not as obvious, the 580 nm band likely becomes more intense as well, although its increased intensity may be masked by the decrease in absorption related to scattering. Note that while the 880 nm band does increase after heating, this change is not significant enough to make it more intense than the band at 580 nm.
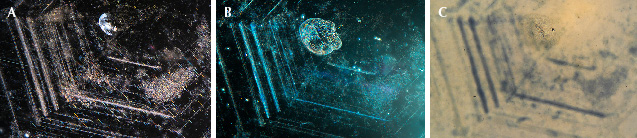
Microscopic observation reveals significant alteration of the rutile silk upon heating. Before heating, the silk was composed of a mixture of long, medium, and short needles and particles arranged in a hexagonal pattern (figure 48A). After heating, the long and medium silk are completely decomposed and the hexagonal clouds of particles are made up of very fine, dusty particles (figure 48B). Also notable is the small inclusion in the core with the concentrically aligned decrepitation halo (figure 48A, top center). After heating, the decrepitation halo has become filled with some residual material, likely from the melting or alteration of the originally intact central inclusion. Finally, the most conclusive evidence of heat treatment comes from comparing the distribution of partially dissolved silk using a fiber-optic light and blue patches of coloration using diffuse transmitted light (figure 48C). The correlation between the remnant silk clouds and the blue zones of color allows for easy identification of heat treatment in this case.
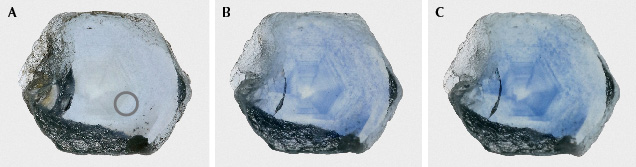
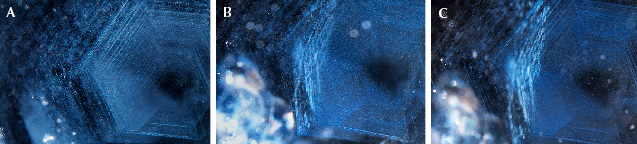
Example 4: Effect of Cooling Rate. While the precise heating conditions for the above experiments are not known, additional tests have been carried out at GIA’s experimental heat treatment facility. In the first experiment, a cloudy, pale blue stone with significant rutile particles and silk was heated at 1700°C for 12 hours in a reducing atmosphere composed of 0.33 atm partial pressure of H2 and 0.67 atm partial pressure of CO2. The sapphire was cooled slowly at a rate of 4°C/minute. This was done twice to ensure the sapphire reached a steady state at these conditions. The same sapphire was heated again at the same conditions but quenched rapidly by placing it in a crucible affixed to an alumina rod that can be retracted from the bottom of the muffle tube. In this way, the sapphire is cooled from 1700°C to about 1000°C in 1–2 minutes and then further cooled to room temperature in roughly 15 minutes. The spectra and photos of the sapphire after various stages of heat treatment demonstrate the deeper blue color of the rapidly cooled sapphire compared to the slowly cooled heating (figures 49 and 50). When the sapphire is slowly cooled, the titanium dissolved into the corundum can start to exsolve as rutile (TiO2) as the solubility of titanium in corundum decreases with decreasing temperature. As the rutile particles precipitate and grow during cooling, the titanium exits the corundum lattice, causing a reduction in the Fe2+-Ti4+ intervalence charge transfer band and a reduction in the blue coloration. Rapid cooling quenches in the high-temperature solubility of titanium in the corundum lattice and retards exsolution of rutile upon cooling. Note, however, that rapid cooling does not lead to complete dissolution of rutile silk and particles in the sapphire, as seen in the photomicrographs in figure 51. The use of intense fiber-optic lighting shows that even the rapidly cooled sapphire has an abundance of light-scattering rutile particles. The presence of these rutile particles suggests either that rutile was never fully dissolved into the corundum lattice at 1700°C or that even with rapid cooling there was still some very rapid exsolution of rutile.
Example 5: Creation of Trapped-Hole Coloration. For one final example, we consider two sapphires with entirely different responses to heat treatment. Many Montana sapphires are heated to create or intensify yellow or orange color by creating what is referred to as a “trapped hole.” This trapped-hole chromophore involves a Mg2+ atom substituting for Al3+ with a missing electron on a nearby oxygen anion for charge balance (a trapped hole). This leaves the oxygen anion with a charge of –1 and causes it to absorb light at 450 nm, creating intense yellow or orange color (see Dubinsky et al., 2020). Some heaters who specialize in treating Montana sapphire will carry out what is referred to as a “fancy burn” in an oxidizing atmosphere to bring out yellow and orange hues. The creation of trapped-hole absorption centers by heating Montana sapphires in an oxidizing environment was carefully documented by Emmett and Douthit (1993).
Many heated fancy-color sapphires will display an intense yellow or orange core where the trapped-hole chromophore is developed. This heating needs to be carried out in an oxidizing environment in order to create a trapped hole. Heating in reducing environments can facilitate the charge balance of Mg2+ by oxygen vacancies instead of the trapped hole on the O– anion.
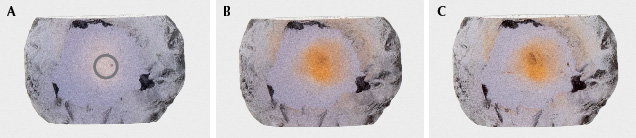
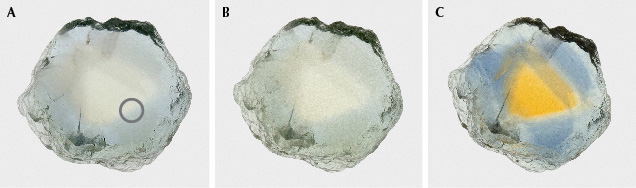
Figures 52–55 show the results of an oxidizing heat treatment run on two sapphires, one from Rock Creek (figures 52 and 53) and one from Dry Cottonwood Creek (figures 54 and 55). Both were heated once at 1200°C in a pure O2 atmosphere for 12 hours, then heated again at 1700°C in a pure O2 atmosphere for 12 hours.
The sapphire from Rock Creek developed an orange core after heating in O2 at 1200°C (figure 52). The UV-Vis spectrum shows this change, with an increase in absorption in the region from 350 to 500 nm (figure 53). The difference between the heated and unheated spectra shows the creation of the trapped-hole absorption band centered at around 480 nm. The negative dip in the difference spectrum centered at about 580 nm indicates that heating also reduced the Fe2+-Ti4+ intervalence charge transfer absorption. Heating at 1700°C resulted in a negligible change in color, possibly with a slight increase in the trapped-hole absorption band in the UV-Vis spectrum.
The sapphire from Dry Cottonwood Creek did not develop the trapped-hole chromophore after heating at 1200°C (figure 54). The only change at this lower temperature was a lightening of the blue color due to a reduction in the Fe2+-Ti4+ IVCT absorption (figure 55). The trapped-hole chromophore is only developed in this sample by heating at the higher temperature of 1700°C in pure O2 (figure 55). This sapphire also developed blue color in its rim during heating due to dissolution of rutile particles and introduction of Ti4+ cations into the corundum structure, leading to creation of Fe2+-Ti4+ IVCT chromophores.
The fundamental difference in the behavior during the oxidizing heat treatment is in the way excess Mg2+ cations are charge-balanced in the original unheated sapphire. The FTIR spectrum of the Rock Creek sapphire here contained the acceptor-dominated 3000 cm–1 series (the “Punsiri band”), which is caused by H+ cations charge-balancing excess Mg2+ cations. This hydrogen is easily burned away at relatively low temperatures (i.e., 1200°C), leaving the Mg2+ to be charge-balanced by a trapped hole when heated in an oxidizing environment (J.L. Emmett, pers. comm., 2022). The Dry Cottonwood Creek sapphire did not have the acceptor-dominated 3000 cm–1 series in its FTIR spectrum, indicating that any excess Mg2+ would have been charge-balanced by oxygen vacancies. Trapped holes in this case can only be created by diffusion of aluminum vacancies into the sapphire to annihilate oxygen vacancies, thereby allowing Mg2+ to be charge-balanced by trapped holes instead of the oxygen vacancy (J.L. Emmett, pers. comm., 2022). But diffusion of aluminum vacancies requires extreme temperatures and long heating times, which is why this is only seen in the sapphire heated at 1700°C for 12 hours and not when heated at the lower temperature of 1200°C. Only a small number of Montana sapphires have been heated this way at GIA’s experimental heat treatment facility so far. However, in many years of heat treating Montana sapphires from all three secondary deposits, Dr. Emmett has observed significant differences in the way sapphires from these deposits react to oxidizing heat treatment. Missouri River sapphires do not have the acceptor-dominated 3000 cm–1 series in the infrared and therefore require high temperatures in oxidizing conditions to develop trapped-hole coloration. On the other hand, Rock Creek sapphires often have the acceptor-dominated infrared features at 3000 cm–1 (see figure 23) and frequently react well to an oxidizing fancy burn at temperatures as low as 1200°C. According to Dr. Emmett, a small proportion of Dry Cottonwood Creek sapphires have the acceptor-dominated 3000 cm–1 features and will develop yellow or orange color at 1200°C, but more frequently these require higher temperatures around 1700–1800°C.
CONCLUSIONS
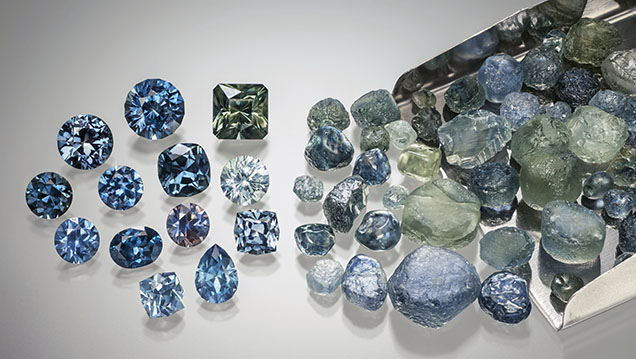
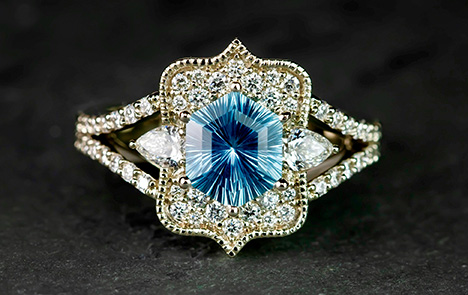
With large volumes of Montana sapphire being produced in consistent and reliable quantities (figure 56), the secondary Montana sapphire deposits are some of the most important American gem mines in modern times. The material fits a wide variety of market demands, from heated goods under 1 carat size to rare, fine, unheated sapphires up to several carats. Aggressive marketing by Potentate Mining and its affiliates as well as by independent artisanal miners, especially through social media, has driven up interest in these gemstones both domestically and in the international gem and jewelry market. Identifying the origin and treatments for Montana sapphires can be relatively straightforward based on inclusions and trace element chemistry, allowing the provenance of these stones to be preserved and traced once the stones enter the gem and jewelry market. With the wide range of colors produced, Montana sapphire is an exceptionally versatile gem, finding a home in nearly any style of jewelry (figure 57). With large reserves remaining at Rock Creek, Missouri River, and Dry Cottonwood Creek and their growing popularity with consumers, Montana sapphire is likely to play an increasingly important role in the market.