Methods and Challenges of Establishing the Geographic Origin of Diamonds
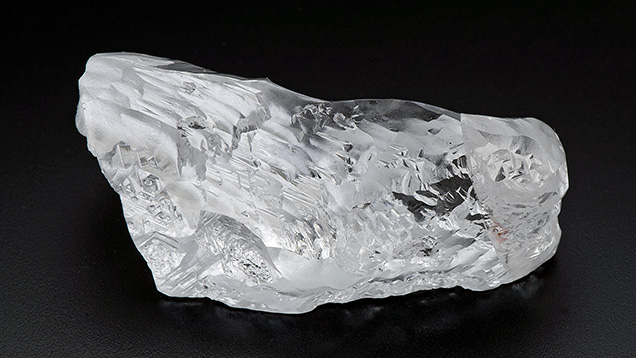
ABSTRACT
There is growing interest in developing methods to deduce the geographic origin of diamonds. Most approaches have focused on trace elements within diamonds, which can be sensitive recorders of geological conditions during the growth of minerals. Gem-quality diamonds have ultra-low concentrations of trace elements, making them extremely challenging to analyze quantitatively. Nonetheless, high-quality trace element data from multiple studies reveal complex and variable patterns, but with striking similarities and overlap between worldwide deposits. Diamond properties such as trace element or isotopic characteristics vary as a function of geological conditions that are not necessarily distinct and resolvable between diamonds of different geographic origin. We conclude that there has been no study by any method demonstrating unique and measurable characteristics that would allow for independent provenance determination of a random individual diamond. For now and the foreseeable future, the only definitive method to establish diamond origin depends on preserving and retaining origin information from the time of mining.
Some famous diamonds, such as the Hope, the Cullinan, and the 404.2 ct “4 de Fevereiro” diamond in figure 1, have high-profile histories that include their geographic provenance. Other diamonds with a known origin can occasionally be sourced in the marketplace or directly from miners. But for the majority of diamonds, this information is not preserved, instead becoming obscured as they move through the supply chain. To some extent, diamond provenance has not been seen as a valuable piece of information and is not presented as a relevant pricing factor the way it sometimes is for some other gemstones. Rough diamonds are typically bought and sold in lots or parcels based on physical characteristics, including color, clarity, size, and morphology. In assembling these parcels, it is common practice to mix diamonds of similar character from different mines. Faceted diamonds are sold mainly on the basis of the 4Cs (color, cut, clarity, and carat weight) and may be mixed further.
Beyond the historical or scientific interest in provenance, several recent developments have put diamond origin at the forefront of conversations in the industry and among consumers. Initially, this was driven by a need to track conflict diamonds and prevent their entrance into the trade. Now, with mounting global sustainability efforts across all industries, consumers are interested in knowing the origin and impact of the goods they purchase. These social pressures have prompted direct efforts, by both privately and publicly funded research groups, to search for distinct geographic signatures among diamonds (e.g., Watling et al., 1995; McNeill et al., 2009; Dalpé et al., 2010; Rege et al., 2010; Coney et al., 2012; Melton et al., 2012; Brill et al., 2020; McManus et al., 2020).
BOX A: COMPARISON OF DIAMONDS TO OTHER GEMSTONES |
Methodologies for geographic origin determination of rubies, emeralds, and other gem materials cannot readily be applied to diamonds. This is due to fundamental differences between diamonds and other gem materials. Diamonds form in the mantle, much deeper than most other gemstones. Except for some mantle-derived olivine/peridot, most other gemstones form in the earth’s crust at relatively shallow depths. Minerals in the crust are the product of multiple melting and differentiation processes that over time have created the crust. These processes concentrate certain elements (known as incompatible elements), which tend to partition into magma as rocks melt. Consequently, crustal rocks have relatively high concentrations of these otherwise rare incompatible elements. When gemstones form within crustal rocks, they naturally inherit higher concentrations of incompatible elements. Furthermore, the crust has great diversity in chemical composition, in contrast to the mantle’s more uniform composition. Because of the chemical diversity of different geological environments in the crust, gemstones formed in different crustal environments often have markedly different trace element compositions. For example, rubies can exhibit differences in certain trace elements (magnesium, titanium, vanadium, iron, and gallium) based on their formation in igneous (basaltic and lamprophyric), metamorphic (granulitic and metalimestone), and metasomatic (skarn and pegmatitic) environments (Palke et al., 2019b). In some cases, it is possible to relate specific compositional features in these gemstones to the compositions of known and exposed crustal rocks in the different geologic settings. Determining trace element concentrations within crustal gemstones allows constraints to be placed on the geological setting they formed in, potentially allowing their geographic origin to be inferred. However, distinguishing crustal gemstones that are from the same kind of geological setting but from different geographic locations remains challenging. Multiple layers of evidence, including geochemical and inclusion characteristics, often must be combined in order to better assess a gemstone’s origin. This challenge is even more pronounced for diamonds. Diamonds formed in the same kinds of geological settings are found spread across many different geographic locations. The majority of diamonds form in the thick and ancient portions of the continental lithospheric mantle. There are three main host rock types—peridotite, eclogite, and websterite—that are composed primarily of different proportions of only four minerals: olivine, orthopyroxene, clinopyroxene, and garnet. Compared to crustal rocks, the rocks of the mantle have limited mineralogical and chemical variability. Furthermore, the covalently bonded diamond lattice is extremely compact and regular, composed of only one major element, and cannot easily accommodate the addition of substitutional and interstitial impurity elements. Only a few elements, such as hydrogen, boron, nitrogen, silicon, and nickel, fit into the diamond lattice. Other trace elements in diamond are thought to be present as nano-inclusions of fluid rather than occupying discrete crystal lattice sites (Melton et al., 2012; Krebs et al., 2019). Consequently, as a diamond grows, it incorporates extremely low levels of trace elements compared to other minerals. Nitrogen, however, is a notable exception. Nitrogen is the most common impurity in natural diamond. It can substitute for carbon in the diamond lattice, typically at concentrations of tens to hundreds of parts per million (ppm). Diamond has a median nitrogen value of 160 ppm, with 99% being <1400 ppm (Stachel, 2014). It can occur as isolated nitrogen atoms (C centers), as aggregated pairs (A centers), or as groups of four atoms plus a vacancy (B centers) (Breeding and Shigley, 2009). Unfortunately, the nitrogen concentration and its degree of aggregation overlap completely among diamonds from around the world and cannot be used to determine geographic origin. |
A common goal has been to develop a database of measurable characteristics to compare diamonds from different sources. Trace element characteristics, in conjunction with other observations, have proven useful for origin determination of other gem materials (see box A). Similarly, trace element analysis using mass spectrometry has been regarded as the most promising approach for diamonds (Watling et al., 1995; McNeill et al., 2009; Dalpé et al., 2010; Rege et al., 2010; Coney et al., 2012; Melton et al., 2012; Brill et al., 2020). One study has also used laser-induced breakdown spectroscopy (LIBS) for this application (McManus et al., 2020). This article will discuss these methods and the challenges they face. The reality at the moment is that there is no scientifically robust method to determine the geographic origin of any given diamond (Dalpé et al., 2010; Cartier et al., 2018; Krebs et al., 2019). All current, reliable, and available means of establishing provenance depend on retaining country-of-origin and/or mine-of-origin information, rather than determining this analytically.
DIAMOND FORMATION AND ITS GEOLOGICAL FRAMEWORK
One of the significant concepts emerging from the past century of diamond research is that there are many different ways diamonds can form, with the pertinent variables being the host rock type, the composition of the diamond-forming fluid or melt, and the depth of formation (lithospheric or sublithospheric) (Shirey et al., 2013). There are distinct varieties of diamond that arise in nature, and, crucially, these varieties do not appear to have unique geographic distributions overall.
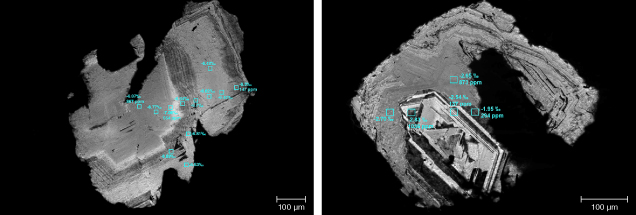
In general, diamonds form as carbon-bearing fluids flow through rocks in the mantle and the carbon crystallizes due to chemical reactions or changes in pressure or temperature. Studying the interior of diamonds with cathodoluminescence has revealed that many diamonds exhibit multiple concentric growth layers, resulting from multiple fluid pulses and episodes of growth (figure 2; Shirey et al., 2013). The layers can have distinct carbon isotope compositions (δ13C), nitrogen concentrations, nitrogen isotope compositions (δ15N) (Stachel et al., 2022b), and perhaps other characteristics as well, such as trace element contents. Diamond trace element and isotopic composition are controlled by the elements present in the parental carbon-bearing fluid, varying with the extent of interaction between the fluid and surrounding host rock minerals (Weiss et al., 2015).
The products of diamond formation in the earth’s mantle are surprisingly similar across many different localities. Categorizing diamonds based on their mineral inclusions provides much of the basis for our understanding of different geological varieties of diamond. The major geological varieties defined by inclusion mineralogy are shown in table 1. These represent diamonds from the lithospheric mantle, formed at approximately 150–200 km, and exclude the rarer (<2%) sublithospheric or “superdeep” varieties of diamonds (Smith et al., 2017; Stachel et al., 2022a).
Diamonds we see at the earth’s surface have been carried up in magmas that form kimberlites (or, less commonly, lamproites). The magma rips up pieces of diamond-hosting mantle rocks hundreds of kilometers below the surface. During this journey, mantle rocks break apart and release diamonds into the magma, forming so-called xenocrysts (crystals foreign to the magma). Individual kimberlite deposits at the surface often contain multiple populations of diamonds mixed together, which could have originally crystallized in completely independent and episodic diamond-forming events within different host rocks. The spatial and temporal scale of diamond-forming events at depths in the mantle is not well understood because it is never exposed. Multiple eruption phases of a kimberlite may sample different portions of the lithosphere. Compiling a complete picture of diamond characteristics from any given deposit means taking into account multiple dissimilar populations. Depending on the nature of the deposit, it could be difficult to judge whether a sample suite of diamonds is truly representative of that deposit. The situation may be more complex for alluvial deposits, which may contain diamonds eroded from multiple primary known or unknown kimberlite/lamproite sources.
The known geological varieties of diamond, including the major categories outlined in table 1, are not geographically restricted. Diamond properties are a function of geology, not geography, and the geological controls for the most common varieties of diamond result in broad, overlapping similarities between many geographic settings. For example, the most well-studied diamonds, found in most deposits around the world, formed within harzburgite (a type of peridotite) host rocks, at comparable pressure and temperature conditions, from carbon-bearing fluids of similar composition (Shirey et al., 2013). These are the kinds of diamonds that contain rare vibrant purple Cr-pyrope garnets. The diamond exploration strategy is to search for these garnets and other indicator minerals released by fragmentation of diamond-hosting rocks and dispersed in surficial sediments. The success of the strategy is underpinned by the fact that the predominant diamond formation processes are often similar from one deposit to the next worldwide. Searching for kimberlitic indicator minerals with the same composition as those from existing mines is an effective technique for finding new diamond-bearing kimberlites (Kjarsgaard et al., 2019).
Physical Characteristics and Traditional Gemological Observations. Each diamond deposit encompasses a range of diamond morphologies, surface textures, colors, and other characteristics. Some localities have more visually distinctive rough diamonds than others, which is noticeable upon examining parcels of diamonds from that locality. For example, the Ellendale mine in Australia produced a large proportion of resorbed, smooth-surfaced dodecahedral (or tetrahexahedral) yellow diamonds (Hall and Smith, 1984; Jaques et al., 1986). The Marange alluvial deposit in Zimbabwe has diamonds with a nontransparent coating and radiation damage (Smit et al., 2018), while the Victor mine in Canada has produced a high proportion of well-formed, near-colorless octahedral crystals with few mineral inclusions (“The real value of Victor Project,” 2007).
Of course, these are not the only examples of particular features associated with certain diamond localities. An unusual example is the porous, micro-polycrystalline diamond variety known as carbonado. Although not of gem quality, it is interesting because it is only recovered from placer deposits in Brazil and the Central African Republic (Heaney et al., 2005). As another example, the Cullinan mine in South Africa is known as a chief source of boron-bearing, type IIb diamonds (King et al., 1998). A random type IIb diamond circulating in the marketplace has a reasonable probability of being from Cullinan. The Argyle mine in Australia, which closed in 2020, was a leading producer of pink (and many brown) diamonds. Argyle’s pink diamonds generally have highly aggregated nitrogen (more B centers than A) and a color that is associated with slightly more diffuse pink graining compared to pink diamonds from most other localities, which tend to have less aggregated nitrogen and a pink color associated with sharply defined glide planes (Gaillou et al., 2012). Argyle was also an almost exclusive source of rare blue to violet diamonds whose color is related to hydrogen (Eaton-Magaña et al., 2018). The distinctive characteristics described here are helpful observations in terms of inferring the origin of some specific diamonds or representative and unmixed parcels, but they are certainly not definitive. Experienced professionals can reach an educated guess about the origin of diamond parcels, or even an individual diamond, but this judgment is highly subjective and cannot be easily verified. For the majority of polished or rough diamonds, there are no distinct characteristics that reveal the geographic origin.
Carbon and Nitrogen Characteristics. Carbon and nitrogen might seem to be a good way to fingerprint diamonds, given their high abundances and isotopic variations in this material. Such analyses could be performed on most diamonds. However, nitrogen abundance variations (measured by infrared absorbance spectroscopy or even imaged with luminescence-based techniques) reveal that the nitrogen content can vary greatly between the growth zones of each diamond (figure 2), not to mention on the scale of millions of diamonds in a single mine.
This variation in nitrogen content is due to the influx of different fluid pulses during diamond growth, each of which may have had a different nitrogen content. Diamonds with an internal tenfold difference in nitrogen content have been documented through secondary ion mass spectrometry (SIMS), a technique that can obtain spatially resolved measurements (see the measurement locations superimposed in figure 2) (Stachel et al., 2022b). These differences are averaged when using bulk analytical techniques such as infrared spectroscopy. Nitrogen abundance variability (within the limited range of possible concentrations) is so pervasive and occurs on such a small scale that nitrogen content alone is not a useful tool to distinguish diamonds from different geological environments.
Both carbon and nitrogen have two stable isotopes (12C, 13C and 14N, 15N), with the lighter atomic mass isotope of each element (12C and 14N) comprising ~99% of the isotopic composition of the respective element. Similar to nitrogen content, the entire global range of both δ13C and δ15N (the part per thousand variation of the 13C/12C and 15N/14N ratios, with respect to a standard) may be represented within one deposit (figures 3 and 4). The main mantle range of δ13C is centered around –5‰, and the global δ13C distributions of diamonds that form in the two main host rocks—peridotite and eclogite—have modes that overlap with this mantle range (figure 4). Consequently, the stable isotope composition of a diamond lends insight into the host rock and fluids from which the crystal formed, but it is not a geographically distinct feature. Even if it were moderately helpful for origin determination, the time-consuming and expensive nature of stable isotope analysis, as well as the formation of ablation pits, makes it unsuitable for routine application to faceted diamonds.
BOX B: WHAT ARE TRACE ELEMENTS? |
Most of the rocks and minerals around us are made up of a relatively small selection of elements, including oxygen (O), silicon (Si), aluminum (Al), calcium (Ca), iron (Fe), magnesium (Mg), sodium (Na), and potassium (K), the most abundant elements in the earth’s crust. Just consider the mineral formulas of some common rock-forming minerals: quartz (SiO2), olivine ([Mg,Fe]2SiO4), potassium feldspar (KAlSi3O8), or what is regarded as the most abundant mineral at great depth within the earth, bridgmanite ([Mg,Fe]SiO3). Many elements on the periodic table do not make an appearance as major defining components of minerals and are present only in trace quantities. In geology, the term trace element generally refers to those elements making up less than about 0.1% by weight (1000 ppm) of a mineral, rock, magma, or other system (Shaw, 2006). In a mineral, elements given in the mineral formula are essential structural constituents (Hanson and Langmuir, 1978) that impart distinct properties and make up the majority of the material. These are called major elements. The terms major and trace elements can therefore refer to different elements depending on the material in question. Diamond is rather special, being composed solely of one major element, carbon. Other examples are gold, silver, and copper, all of which occur in their native form. In diamond, carbon is the only major element, whereas all other elements in this mineral are only present in trace quantities, often expressed in parts per million (ppm), billion (ppb), trillion (ppt), and so on, by weight. The concentrations and relative abundances of trace elements can provide information about the way minerals formed. Trace element analysis of diamond has primarily focused on unraveling the geological settings and elements involved in diamond formation, but a secondary goal has been the forensic/gemological application of trying to distinguish geographic origin. The trace elements typically discussed in the context of diamonds include Cs, Rb, Ba, Th, U, Pb, Ta, Nb, La, Ce, Pr, Sr, Nd, Sm, Hf, Zr, Eu, Ti, Gd, Tb, Dy, Y, Ho, Er, Yb, and Lu, as well as the lighter elements H, B, N, O, Na, Mg, Al, Si, Cl, K, Ca, Fe, and Ni. |
Trace Element Characteristics. Like any mineral, diamond contains minute amounts of elements other than those stated in its mineral formula (see box B). These so-called trace elements may provide a rich geochemical record of the conditions of mineral growth, potentially revealing differences between different deposits. Trace elements can be present at concentrations from many parts per million (ppm) down to parts per billion (ppb), parts per trillion (ppt), or less, so there are enormous ranges of possible concentrations in natural materials. In transparent, gem-quality diamond, the concentrations of trace elements are extremely low, often in the ppt range, making them especially difficult to measure compared to other minerals. The first pioneering measurements of trace elements in gem-quality diamond were made by instrumental neutron activation analysis (INAA) (Fesq et al., 1973; Bibby, 1982), but the amount and quality of data were limited. It is worth noting that the neutron bombardment of INAA severely damages the appearance of the whole diamond being analyzed.
Later studies have employed laser ablation–inductively coupled plasma–mass spectrometry (LA-ICP-MS), a widely used tool for measuring elemental compositions of many geological materials, including gemstones (Liu et al., 2013). Laser ablation involves vaporizing a small amount of the sample material by blasting a tiny crater into it with a laser. The liberated sample particles are ionized in a plasma to form a beam of atomic and small polyatomic ions, which are then continuously separated by mass/charge in a mass spectrometer (figure 5A). This technique has been applied to many gemstones (see review in Groat et al., 2019), including blue sapphire (Palke et al., 2019a), ruby (Palke et al., 2019b), and emerald, all of which contain abundant trace elements that are relatively straightforward to measure.
When it comes to transparent, gem-quality diamond, however, the ultra-low concentration of most trace elements is problematic. The low bulk concentration of trace elements means that high-quality data cannot be obtained by routine “online” LA-ICP-MS analysis of gem-quality diamond because most elements fall near or below the limit of detection (McNeill et al., 2009). Increasing the laser energy to ablate more diamond in an effort to overcome the low concentration carries the risk of uncontrolled fractionation at the ablation site during sampling and increased diamond destruction. For these reasons, applying routine LA-ICP-MS techniques to gem-quality diamonds yields data that are generally not quantitative and whose uncertainties are difficult to evaluate (McNeill et al., 2009), making them of limited use for investigating diamond paragenesis and potential geographic variability. For example, in a study of 400 monocrystalline (nonfibrous) diamonds analyzed by LA-ICP-MS (Rege et al., 2010), the trace element patterns exhibited strong similarities regardless of geographic locality or geological paragenesis. Early attempts such as this struggled to accurately characterize detection limits or demonstrate that the data produced were significantly different from instrumental background.
Aside from carbon, most elements do not incorporate easily into the diamond crystal lattice as it grows, which is one of the reasons high-clarity diamond crystals have such low trace element concentrations. A few trace elements such as hydrogen, boron, nitrogen, silicon, and nickel (also common in laboratory-grown diamonds) may be incorporated as defects in the diamond lattice. But the majority of trace elements of geological interest, when they do occur in gem diamond, are thought to be hosted as fluid nano-inclusions that are simply too small and sparse to see (Melton et al., 2012; Krebs et al., 2019, 2020).
In contrast to the ultra-low trace element concentrations in gem-quality diamond, a particular growth habit called fibrous diamond has diamond trace element concentrations that are at least two to four orders of magnitude greater (Weiss et al., 2008). Fibrous diamond has a cloudy appearance due to abundant sub-micrometer-sized high-density fluid (HDF) inclusions (Navon et al., 1988) and is generally not considered gem-quality because of the diminished clarity. However, the abundance of HDF inclusions in fibrous diamond causes the bulk trace element contents to be high enough to analyze using LA-ICP-MS. Most trace element studies of diamond have focused on fibrous diamond (Tomlinson et al., 2005, 2009; Zedgenizov et al., 2007b; Rege et al., 2010; Smith et al., 2012; Weiss et al., 2013; Klein-BenDavid et al., 2014). Even though these analyses are based on micro-inclusions hosted in diamond and not the diamond itself, there is evidence that similar trace element characteristics exist at lower concentrations in gem-quality diamond that do not contain visible inclusions (Jablon and Navon, 2016; Krebs et al., 2019). This suggests that fibrous diamonds and their HDF inclusions are broadly informative of nonfibrous, gem-quality diamonds and provide an additional basis for discussing the prospect of origin determination (see the section “Fibrous Diamonds Bolster Our Insight into Trace Elements”).
BOX C: “OFFLINE” LASER ABLATION TECHNIQUE |
This specialized method for measuring trace elements in diamond involves a pre-concentration step that allows a larger sample to be collected in a controlled fashion. It was developed by McNeill, Pearson, and colleagues at Durham University specifically for analyzing diamond (McNeill et al., 2009). The diamond is placed in an enclosed vessel with a window through which the laser can pass. Ablation takes place within this sealed vessel, allowing the ablated material to accumulate over the course of minutes or even hours. Compared to the direct uninterrupted ablation-to-analysis of LA-ICP-MS, this offline sampling technique means a much larger amount of diamond, and therefore a larger sample of trace elements, can be ablated in a controlled way. The accumulated sample is then taken up in acid and analyzed by conventional solution-based ICP-MS. Analyzing solutions greatly simplifies standardization, circumventing the need for a diamond standard. Appropriate solution standards with elemental concentrations similar to that expected in diamond can easily be prepared. Weighing the diamond before and after ablation gives the mass of diamond ablated, providing a way to recast results as elemental concentrations within the diamond. Offline ablation allows much higher volumes of analyte to be pre-concentrated before measurement rather than having the ablated material swept directly into a gas stream for analysis, as in LA-ICP-MS, effectively boosting what is known in analytical work as the signal-to-noise ratio by several orders of magnitude. To measure a signal, having a strong signal and having low background noise are equally important. An example of low signal-to-noise ratio occurs when the noise from city lights prohibits the signal of distant stars from being seen by curious stargazers. In rural areas, background light is so low that even faint signals from stars can be seen. In analytical work, scientists must pay special attention to the relative contributions of signal and noise. The limit of detection (LOD) provides a threshold level where weak signals cannot be confidently distinguished from background noise. One definition of the LOD is 3× the standard deviation of the blank/background (Currie, 1968). However, we want to know how much of the element there is, not just that it can be detected. For the exceptionally low trace element contents of most gem-quality diamonds, which may be only marginally above the background noise, McNeill et al. (2009) argued that a more stringent limit is needed: the limit of quantification (LOQ). This is defined as 7 to 10× the standard deviation of the blank/background (Currie, 1968), and it is a better cutoff to ensure that the signal seen above the background can be used to calculate element concentration. Data must exceed the LOQ (the minimum accurate quantifiable value) to be considered truly quantitative. A number of studies have reported conventional online laser ablation results from gem-quality diamonds but with such low signal-to-noise ratios that LOQ criteria could not be met (Rege et al., 2005, 2010; Coney et al., 2012; Brill et al., 2020). If measurements do not exceed the LOQ, they may carry qualitative meaning, but large uncertainties will obscure comparison between samples, restricting their utility. Offline laser ablation with ICP-MS enables quantitative trace element data for gem-quality diamond. However, the technique is not without drawbacks. The amount of diamond that must be ablated is large compared to the typical ablation crater involved in conventional LA-ICP-MS of other materials. For example, analyses of most colored gem materials using LA-ICP-MS would involve a spot size of 50–100 μm, meaning that an analysis can be performed on the girdle of a valuable ruby, sapphire, or emerald and would not be visible to the unaided eye. For offline ablation ICP-MS of diamond, larger raster patterns of 500–500 μm are typical, extending several hundred micrometers deep into the diamond. In addition to being (partially) destructive, the technique is slow. Whereas ablation and analysis in conventional LA-ICP-MS might extend for 40–180 s, offline ablation for diamond normally requires up to 4 hours (and in some cases up to 20–30 hours). More time is needed to carefully clean the diamond with ultra-pure acids prior to ablation, to prepare the ablated sample into a solution, and ultimately to complete the ICP-MS analysis. A high-tech and expensive clean lab is necessary to minimize contamination. All samples are processed in parallel with total procedural blanks so that the combined background (or noise) of trace elements present in all acids and materials involved can be measured. The stringent requirements are such that only two laboratories have done this rigorous level of analysis for gem-quality diamond, first at Durham University and later at the University of Alberta by Pearson and colleagues. |
Returning to the more challenging analysis of gem-quality diamond, there is a modified laser ablation technique that can achieve superior results. McNeill et al. (2009) developed a sample pre-concentration technique involving “offline” laser ablation (see box C; figure 5B). This technique was developed as an improvement over conventional “online” LA-ICP-MS, specifically to measure trace elements and some radiogenic isotopes in gem-quality diamond (McNeill et al., 2009; Klein-BenDavid et al., 2010). Part of the aim in developing this advanced methodology was to investigate whether trace elements can be linked to specific geographic origins (McNeill, 2011).
The offline laser ablation sampling technique permits meaningful quantitative measurement of trace elements in even the purest gem-quality diamonds, although it currently requires a substantial input of time, laboratory infrastructure, and analytical expertise. This method is also moderately destructive, further barring its routine application to faceted gem diamonds. It involves ablating a relatively conspicuous “pit” on the order of 500 μm wide and several hundred micrometers deep. Only a limited number of diamond localities have been studied so far: 11 from Brazilian sublithospheric diamonds (Timmerman et al., 2019a); 10 from Koffiefontein, Letlhakane, and Orapa (Timmerman et al., 2019b); one from Udachnaya; one from Mir; one from Venezuela; 10 from Cullinan (McNeill et al., 2009); 24 from Finsch, Newlands, and Victor (Krebs et al., 2019); and 10 from Akwatia, Ghana, and the De Beers Kimberley Pool, South Africa (Melton et al., 2012). In small sample sets, statistical analysis of variance and logistic regression suggests significant differences between populations (e.g., McNeill, 2011), but these differences appear less distinct upon analysis of a wider variety of diamonds. Differences may be apparent when considering averaged trace element patterns from different localities, or even diamonds with sulfide versus silicate inclusions from a single locality, but comparing individual diamonds shows considerable overlap from one deposit to the next (Krebs et al., 2019). As discussed by Melton et al. (2012), some of these measurements may be skewed by the intersection of small mineral inclusions during the course of ablation, which may or may not be avoidable depending on the sample. This possibility requires additional care during ablation and later during interpretation.
Although the data are limited, a key outcome of this work is the finding that many gem diamonds have trace element patterns comparable to those of fibrous diamonds (Krebs et al., 2019; Timmerman et al., 2019b). In support of such a connection, HDF inclusions similar to those in fibrous diamonds have been reported within nonfibrous gem-quality diamonds (from the Voorspoed and Venetia mines in South Africa, for example) trapped along the twinning planes of macles (Jablon and Navon, 2016). These results allow us to draw on the existing literature on fibrous diamonds in the discussion of trace element use for geographic origin determination.
FIBROUS DIAMONDS BOLSTER OUR INSIGHT INTO TRACE ELEMENTS
There are marked similarities between fibrous diamonds and gem-quality nonfibrous diamonds upon comparing the major element compositions of fluid inclusions and trace element compositions of bulk diamond (Jablon and Navon, 2016; Krebs et al., 2019). The similarities suggest that the more extensive work on trace elements in fibrous diamonds also offers insight into gem-quality diamonds. Fibrous diamond trace element properties cannot be claimed as a proxy for all diamonds, as some diamond varieties are thought to be formed from distinctly different fluids, such as methane-bearing reduced fluids (Smit et al., 2016, 2019) and metallic Fe-Ni-C-S liquids (Smith et al., 2016, 2017). However, the connection between fibrous diamonds and transparent, nonfibrous diamonds formed in the lithospheric mantle applies to many mined gem-quality diamonds derived from the lithospheric mantle.
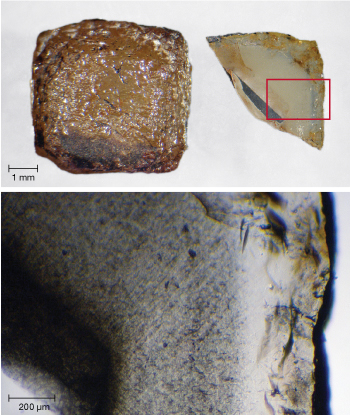
Fibrous diamonds are fluid-rich diamonds that trap small droplets of the diamond-source fluid as inclusions during growth. They have a dendritic single-crystal growth habit (like a snowflake) that grows as a set of crystallographically controlled, space-filling branches in three dimensions, typically producing an overall cuboid shape (figure 6). The chemical composition of HDF inclusions in fibrous diamonds has been measured by several methods, with electron probe microanalysis (EPMA) being the most common. HDF compositions can be described in terms of four end members (figure 7). There is a silicic end member rich in Si, K, Al, Fe, and water; a low-Mg carbonatitic end member rich in Ca, Fe, K, Mg, and carbonate; a high-Mg carbonatitic end member rich in Mg, Ca, Fe, K, and carbonate; and a saline end member rich in Cl, K, Na, water, and carbonate (Navon et al., 1988; Izraeli et al., 2001; Tomlinson et al., 2006; Klein-BenDavid et al., 2007; Weiss et al., 2009). Compositional variability between end members defines a transitional array bridging the silicic and low-Mg carbonatitic end members. The well-populated compositional array between silicic and low-Mg carbonatitic end members may be explained in terms of near-solidus melt compositions with varying H2O/CO2 ratios in eclogite host rocks leading up to diamond formation (Elazar et al., 2019). In contrast, the saline and high-Mg end members are more detached in compositional space. Only a few diamonds exhibit transitional compositions between saline and high-Mg carbonatitic end members (Klein-BenDavid et al., 2006) as well as between saline and silicic end members (Tomlinson et al., 2006; Weiss et al., 2009).
Trace element measurements by mass spectrometry, with both traditional LA-ICP-MS and offline laser ablation ICP-MS, reveal high degrees of trace element enrichment in the fluids. Trace element patterns define two major groupings: one that is highly irregular, with prominent enrichments or depletions of particular elements, and another that is less irregular (figure 8). Specifically, the more irregular group has depleted compositions of alkali (e.g., K, Rb, Cs) and high field strength elements (e.g., Ta, Nb) and enriched large ion lithophile element concentrations (e.g., Ba, U, Th), while the less irregular group has lower large ion lithophile element concentrations (Tomlinson et al., 2009; Weiss et al., 2009, 2013; Klein-BenDavid et al., 2010; Smith et al., 2012;). The more irregular patterns are thought to relate to fluid interaction with the subcontinental lithospheric mantle, while the less irregular patterns may be a signature of the convecting mantle beneath the lithosphere (Weiss et al., 2013).
By combining trace elements with strontium isotope analyses, the origin of the saline fluid end member has been linked to the involvement of subducted oceanic crust altered by seawater, because seawater is known to have a very enriched 87Sr/86Sr isotope composition (Weiss et al., 2015). Furthermore, chemical evolution of such a fluid percolating through peridotitic and eclogitic rocks in the lithosphere is proposed to account for the compositional ranges of carbonatitic and silicic fluids (Weiss et al., 2015). High-Mg carbonatitic fluids may result from near-solidus melting in peridotite, while silicic-to-low-Mg carbonatitic fluids may reflect melting in eclogite (Tomlinson et al., 2009; Weiss et al., 2009, 2011; Klein-BenDavid et al., 2014; Elazar et al., 2019). Fibrous diamonds therefore provide a key signal of the input of subduction-derived seawater fluids for fibrous diamond growth. These fluids may also lead to metasomatism of the lithospheric mantle (Miller et al., 2014; Weiss et al., 2015).
Fibrous diamonds and their purer gem-quality diamond counterparts therefore reveal two overarching trace element patterns, thought to reflect two major geological processes governing their formation in the mantle. Measurements in both fibrous and gem diamonds show that diamonds from different deposits can have similar patterns. For example, a striking similarity in trace element patterns has been found between suites of fibrous diamonds from two different Canadian deposits: the Wawa area of Ontario and the Ekati mine in the Northwest Territories (Smith et al., 2012). These findings suggest it might not be uncommon to encounter instances where complete overlap precludes an origin determination on the basis of trace elements alone. Conversely, diamonds from the same deposit can have dissimilar patterns, which is not surprising given the fact that many deposits contain multiple diamond populations with geologically distinct sources in the mantle.
Laser-Induced Breakdown Spectroscopy. As a comparatively new analytical technique applied to diamond, LIBS might offer an alternative way to capture chemical characteristics. It involves focusing a rapidly pulsed laser (the same kind as used for LA-ICP-MS) onto the sample to form a plasma and analyzing the spectrum of light emitted to reveal the characteristic wavelengths from elemental and molecular emissions (figure 5C; Senesi, 2014; Harmon and Senesi, 2021). The laser spot size is normally tens to hundreds of micrometers in diameter. Typical detection limits for most elements are in the tens of ppm range (Harmon and Senesi, 2021), but modified techniques such as double-pulse LIBS or nanoparticle-enhanced LIBS can potentially increase the signal-to-noise ratio and lower the detection limits (Koral et al., 2018).
LIBS has a wide range of applications and has been used previously to characterize major and trace elements in a variety of gemstones (Rossi et al., 2014), including efforts toward origin determination of ruby and sapphire (Kochelek et al., 2015). For gem-quality diamonds, most element concentrations fall well below LIBS detection limits. Nevertheless, each spectrum captures a complex combination of signals whose peak shapes and positions are affected by many variables.
Multivariate statistical analysis can be used to recognize shared or differentiating features among sample suites (Harmon and Senesi, 2021). It is possible to compare entire emission spectra for differences, which can potentially be used to recognize geological and geographical patterns, even if the spectra are not deconvoluted into properties such as trace element concentrations. This is a powerful capability of LIBS spectroscopy. Upon comparing spectra, however, it is crucial to account for the fact that spectra collected in multiple analytical sessions can have significant differences arising from day-to-day changes—for example, in temperature, humidity, laser stability, and so on. To ensure data reliability, for LIBS or any other technique, it should be possible to measure a specimen multiple times, independently, and reproduce the same result.
One pilot study has attempted to use LIBS to distinguish diamonds by their different geographic origins. In this initial study involving multiple suites of 30 natural diamonds each, sourced from 12 different localities, plus two suites of laboratory-grown diamonds, McManus et al. (2020) proposed that it was possible to discriminate between the diamonds from different sources. Variable elemental emissions were observed from H, C, N, Na, Mg, Al, Si, K, Ca, Ti, V, Cr, Fe, Co, Ni, Cu, Sr, Ba, O, Ne, and Ar (the carrier gas). However, these analyses were performed on natural rough surfaces cleaned only with isopropyl alcohol rather than extensive acid leaching (compare with McNeill et al., 2009), which means the LIBS spectra could include surface contamination (that could in itself be origin-specific). Even after apparently ablating down through the surface with successive laser pulses, lingering foreign materials on the diamond surface and in shallow surface-reaching fractures could overwhelm the comparatively scant abundance of trace elements actually inside a diamond.
Regardless, a significant differentiating factor in the McManus et al. (2020) LIBS data lies with molecular C-C and C-N emissions (from the two most abundant elements in diamond, C and N), not just trace element characteristics. It is not clear what information these C-C and C-N peaks contain. Potentially, the C-C and C-N molecular emissions vary as a function of carbon and nitrogen isotope composition. Isotopic shifts in optical features are orders of magnitude greater for molecular emissions than for atomic emissions (Harmon and Senesi, 2021). The C-C and C-N peaks may also vary with nitrogen concentration and nitrogen aggregation state. However, many measurements of these carbon- and nitrogen-related variables from previous studies using other methods do not show geographic distinctions (e.g., figures 3 and 4). Although it is possible that C-C and C-N emissions, perhaps under the influence of trace elements or lattice defects, contain rich and complex information, the specific constituents with the potential to convey geographic differences remain uncertain. It is also unclear how to ensure data quality without first understanding the meaning of spectral features of interest.
Alternatively, the observed C-N peaks reported by McManus et al. (2020) could reflect small and variable contributions from atmospheric nitrogen contaminating the argon gas flowing over the sample surface. This possibility cannot be dismissed, because the study did not include any nitrogen-free diamond samples or standards or measurements of the possible dynamically variable trace nitrogen content of the atmosphere in the ablation chamber. Also, the sensitivity of LIBS to nitrogen in diamond remains unclear. An unrelated study comparing point analyses in different crystallographic sectors of a laboratory-grown diamond found differing C-C and C-N emission intensities that were argued to correlate with different assumed nitrogen concentrations (Lebedev et al., 2020), but again it is difficult to entirely rule out atmospheric influences. Without more details of the analytical routine and data handling, it is difficult to evaluate the results.
As with any spot analysis, LIBS spectra collected from a single point may fail to account for internal heterogeneity. Diamonds often have heterogeneous growth layers in terms of nitrogen concentration, nitrogen isotope composition, and carbon isotope composition that may lead to non-uniform C-C and C-N emissions. The pilot study of McManus et al. (2020) does not appear to have explored spatial variations within samples. In addition to heterogeneity within individual diamonds, it is also important to account for the fact that many diamond mines contain multiple populations with distinct properties. Future studies should attempt to capture these variables.
A further challenge with LIBS is that the spectra contain artifacts specific to the instrument used, meaning spectra collected on a given instrument are best compared against others collected with the same equipment (Harmon and Senesi, 2021). Although LIBS offers many appealing aspects as an analytical tool, such as speed and simplicity, its suitability for evaluating and comparing gem-quality diamonds remains to be demonstrated.
PRESERVING GEOGRAPHIC ORIGIN INFORMATION FROM THE TIME OF MINING
As an alternative to inferring origin based on an independent assessment of diamond properties, finding a way to preserve this information from mine to market offers some advantages. If the origin is retained and certified through documentation or other means, it can be stated as a fact rather than as an inference or opinion, and it avoids the potential problem of multiple localities having diamonds with unresolvable, overlapping physical and geochemical characteristics. Perhaps the greatest advantage is that it is a simple and straightforward approach that can be accomplished with existing tools, albeit with logistical challenges.
There are now several industry initiatives for diamond traceability, given increasing attention to corporate governance, environmental impact, and social responsibility. For example, De Beers has developed a traceability initiative called Tracr that uses blockchain, a secure digital record of transactions, to allow a given diamond to be traced from mine to market. Lucara has its own blockchain traceability with Clara Diamond Solutions. Alrosa has developed a laser nanomark (capable of being read at any time) to identify its rough diamonds in a way that survives cutting and polishing. Rio Tinto developed a traceability program to track diamonds from its Argyle mine (now closed) through the supply chain.
Ultimately, traceability initiatives should encompass diamonds from both large-scale and artisanal miners. There is arguably a need for independent verification of origin information by third parties not involved in the sale of diamonds. Several such companies offer ways to verify traceability from mines, through the supply chain, including Everledger, using blockchain; diamond technology company Sarine, with Diamond Journey Traceability; and SCS Global Services, through their SCS-007 standard, which involves verifying supply chain documentation as well as collecting chemical information (through a partnered laboratory using LA-ICP-MS). GIA also provides a provenance service called the Diamond Origin Report, which matches the physical and spectral characteristics of a polished stone with that of a previously submitted rough diamond from a disclosed locality. More recently, the Institute launched the GIA Source Verification Service, which verifies origin information using supply chain documents and invoices from vetted manufacturers and provides this information through its online GIA Report Check service.
This level of investment in traceability by multiple stakeholders is a promising step forward. Preserving the geographic origin information is an exciting prospect not only for consumers, but also for diamond geologists. Just like fossils, meteorites, and mineral specimens, diamonds have a greater value to the consumer and to the scientist when they can be tied back to a specific community, geological setting, age, or process.
CONCLUDING REMARKS
Relative to mantle-derived diamond, gem minerals that formed in the crust are more amenable to inferring provenance on the basis of trace element analysis. Crustal minerals tend to incorporate higher concentrations of trace elements as they grow, and there is inherently more geochemical variability in crustal rocks (see the G&G Winter 2019 special issue on origin determination for colored gemstones). For example, trace elements have been widely employed with reasonable success for discriminating the mine of origin for ruby and sapphire (Saminpanya et al., 2003; Abduriyim and Kitawaki, 2006; Peucat et al., 2007; Pornwilard et al., 2011; Harlow and Bender, 2013; Sutherland et al., 2015). But even under these more favorable circumstances, when two different localities offer similar geological settings there can still be problematic overlap in trace element characteristics, such as for deposits of marble-type ruby and metamorphic blue sapphire (Krebs et al., 2020).
The recognized and resolvable varieties of natural diamond based on all measurable properties correspond to geological variables such as host rock type, the pressure and temperature conditions of crystal growth, and the composition of diamond-forming fluids. Generally these resolvable varieties, such as gem-quality lithospheric peridotitic or eclogitic diamonds, are not restricted to a single geographic origin. Instead, they occur across multiple deposits on a global scale, and multiple varieties can be observed within a single deposit.
Diamonds from different deposits can have indistinguishable trace element characteristics, and diamonds from the same deposit can have marked dissimilarities. The underlying patterns that have been recognized within trace element data are associated with geological processes, irrespective of geographic origin. If there are features unique to individual deposits, they must be subtle features, masked by the more pronounced overarching geological variations that are responsible for characteristics such as inclusion mineralogy or the appearance of enriched versus non-enriched trace element patterns.
Observations to date suggest that trace element analysis may not be a definitive approach for inferring diamond origin. Although current techniques to quantitatively analyze trace elements in diamond are time-consuming, expensive, and somewhat destructive to the sample, these are not the principal hindrances. Even well-controlled, quantitative measurements made using offline laser ablation techniques do not appear to show distinct signatures associated with different mines. In other words, future improvements to methods for measuring trace elements are not necessarily expected to resolve this issue—and even if they could, it might not translate into a feasible routine service for faceted gem-quality diamonds. Importantly, any methodology must be capable of scaling up from preliminary studies involving hundreds of diamonds to high-quality datasets involving hundreds of millions of diamonds in order to be viable for commercial origin determination.
Given the interest and importance of this issue, it may be worth further exploring a combination of techniques using large sample suites. GIA continues to actively investigate this challenge to see whether some level of origin determination is possible, even if the odds of success appear to be low. For now, however, all reliable and rigorous services to establish the geographic origin of a diamond depend on retaining country-of-origin and/or mine-of-origin information from mine to market, rather than attempting determination through independent geochemical analysis.
In short, the absence of a feasible method to accurately determine diamond origin is due to a combination of geological and analytical limitations:
- Diamonds from different mines often form by comparable geological processes in the mantle and have overlapping physical and chemical characteristics.
- Trace element data collected to date, though necessarily limited, show no clear evidence of distinct geographic signatures.
- Even if the initial results were encouraging, the only proven method for quantitative trace element analysis of gem-quality diamond is prohibitively slow, expensive, high-tech, and destructive. These limitations preclude a large global-scale investigation into origin discrimination.