First Identification of Sudoite in Caribbean Ceramic-Age Lapidary Craftsmanship
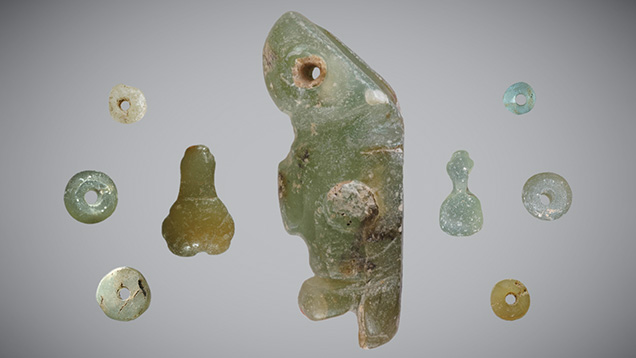
ABSTRACT
Lapidary craftsmanship was an important part of the material culture of Amerindians in the Antilles during the Early Ceramic period (400 BCE to 400 CE). Exhaustive analysis of archaeological beads and pendants from the French islands of the Lesser Antilles has revealed a green lapidary material used for the production of nine artifacts from five archaeological sites: sudoite. This di-trioctahedral member of the chlorite group has the relatively simple chemical formula of Mg2Al3Si3AlO10(OH)8. Previously unknown in sizes suitable for carving, it has never before been identified in any lapidary production and therefore warranted a multi-analytical nondestructive approach to confirm this identification. The analysis was conducted through Raman spectroscopy, FTIR spectroscopy, and X-ray diffraction. The texture and the chemical composition were assessed through SEM-EDS. Color, UV luminescence, and other gemological parameters were studied through standard gemological methods, UV-Vis-NIR spectroscopy, and fluorimetry. Finally, the provenance of the material is considered following a geological approach. The recovery of sudoite artifacts in several archaeological Amerindian sites of the Lesser Antilles supports the already established theory of a pan-Caribbean trade network as old as the first several centuries Before the Common Era.
During the Early Ceramic age, between 400 BCE and 400 CE (Bérard, 2019), a people from northeastern South America appropriated the entire Lesser Antilles, replacing or assimilating the inhabitants of these Caribbean islands (Hofman et al., 2007; Wilson, 2007; Nägele et al., 2020). Indeed, these fishermen-cultivators arrived on the islands with a great innovation: ceramic technology. Several cultural components existed within this period, with a common background called Saladoid, named after the site of Saladero (Rouse, 1992; Bérard, 2019). The inhabitants of the West Indies at this time possessed a complex culture, some characteristics of which are common to the different islands and sub-periods. These include certain ceramic decorations, simple lithic tools, the important use of shells for tools and adornment, and distinctive funerary practices (Bérard, 2013). The lapidary craft, which will be of particular interest to us in this work, is very diversified in terms of both forms and raw materials (Cody, 1993; Murphy et al., 2000; Queffelec et al., 2018; Falci et al., 2020; Queffelec et al., 2020). These materials and artifacts are the subject of various studies examining the supply, distribution, and trade networks of the fishermen-cultivators who inhabited the entire archipelago but whose origins can be traced back to the northeastern part of South America.
An exhaustive study of the ornamental objects of this period recovered from archaeological sites in the French West Indies has revealed a material not mentioned in the classic list of “green rocks” used for the craft: sudoite. This mineral belonging to the chlorite group was named by G. Müller (Müller, 1961; v. Engelhardt et al., 1962) in honor of Toshio Sudo (1911–2000), professor of mineralogy at the University of Tokyo and a pioneer of clay science (Kohyama, 2000). It is a sheet silicate (and thus easy to carve), more precisely a magnesium-rich di-trioctahedral chlorite found mainly in hydrothermal or high-pressure/low-temperature (HP/LT) metamorphism contexts. Its relatively simple chemical makeup, Mg2Al3Si3AlO10(OH)8, does not contain any rare or unusual elements.
Few other chlorite-group minerals have been reported as gems (Bukanov, 2006), and sudoite is not listed in any gemological compilation (e.g., Desautels, 1973; Arem, 1987; Duda and Relj, 1999; Manutchehr-Danai, 2005; O’Donoghue, 2006). It is thus necessary to confirm the mineralogical composition of these nine ornamental objects, initially carried out by Raman spectroscopy and for five artifacts by X-ray diffraction, using a multi-analytical approach that entails nondestructive methodologies applicable to archaeological objects. This mineralogical study will be supplemented by a geological analysis in order to provide a better understanding of the distribution and potential provenance of this rare lithic material.
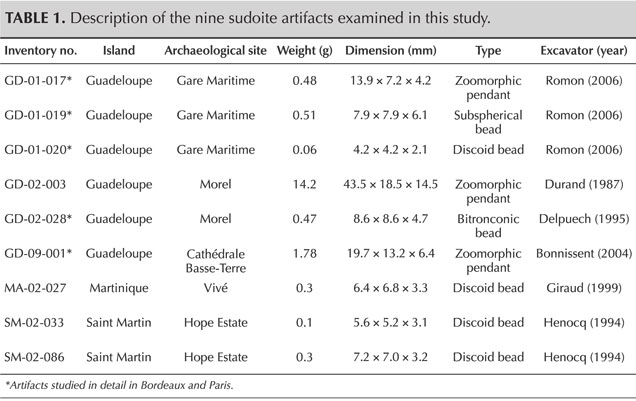
MATERIALS
Archaeological Artifacts. The nine archaeological objects studied in this work were discovered in five archaeological excavations carried out in Guadeloupe, Martinique, and Saint Martin (figure 1 and table 1). A thorough description and the archaeological context surrounding the artifacts can be found in previous publications (Bonnissent, 2008; Queffelec et al., 2018, 2020, 2021). The inventory numbers are taken from the database that records all the lapidary objects from the Lesser Antilles (Queffelec et al., 2021). The inventory codes consist of two letters identifying the island on which the object was found, two digits indicating the archaeological site on this island, and three digits indicating the number of the object in the site. Of these nine artifacts, five were the subject of extensive study in Bordeaux and Paris, while four have only been analyzed on-site using mobile instruments during two study missions to the Antilles (table 1).
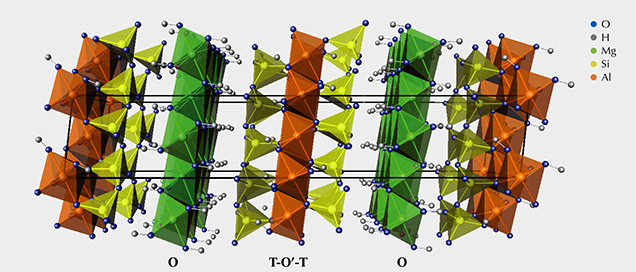
Mineralogy. Sudoite is a phyllosilicate of the chlorite group, with a stacking of sheets (figure 2) formed of T-O'-T layers (T: layer composed of tetrahedra; O’: layer composed of octahedra) separated by O layers (Brigatti et al., 2011). In the T-O'-T layers, the T layers are formed of (Si,Al)O4 aluminosilicate tetrahedra. They enclose a layer of octahedra whose vertices are O2– anions or (OH)– hydroxyl groups, while the octahedral center is occupied by a cation.
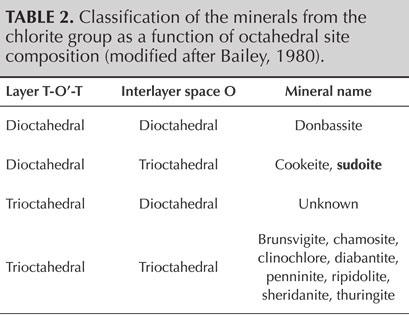
Sudoite, within the chlorite group, is one of the minerals of the di-trioctahedral subgroup (table 2). This implies that the octahedral cationic sites of the T-O'-T sheets are two-thirds (di-) occupied by trivalent cations, here Al3+, creating O' sites of the formula AlO4(OH)2. The octahedral cationic sites of the O sheets are occupied entirely (tri-) by bivalent cations Mg2+, creating O sites of the formula Mg3(OH)6.
This structure and the cationic composition of the octahedral sites lead to a sudoite formula of Mg2Al3Si3AlO10(OH)8. This ideal formula is somewhat different from the formulas derived experimentally, which always contain a variable but not negligible proportion of iron in substitution for aluminum at O' sites (e.g., Billault et al., 2002; Ruiz Cruz and de Galdeano, 2005).
METHODS
With the dual objective of confirming the characterization as sudoite and presenting a first series of gemological parameters for this material, several methods of gemological and mineralogical characterization were deployed on five archaeological objects (table 1), which were temporarily removed to Bordeaux, France. As for the objects that remained in their Caribbean curation site, mineralogical identification relied on portable Raman spectroscopy and basic observation.
The objects were observed with the unaided eye, using a 10× triplet loupe and a Leica Z16APO macroscope, and photographed. Their dimensions were measured with an electronic caliper, and their mass and density were recorded using a hydrostatic balance (Mettler-Toledo XS104) or a conventional balance (Kern TAB 20-3) for objects that remained in the Caribbean. Whenever possible, the refractive index of the objects taken to France was measured using a spot reading method, despite the inherent difficulty of doing so on poorly polished objects. Ultraviolet (UV) luminescence was observed and photographed under short-wave (254 nm) and long-wave (365 nm) UV light (Vilber Lourmat VL-6LC, 6W per tube). We examined the optic character of the more translucent artifacts using a GIA Gem Instruments polariscope.
Various complementary methods were applied to the objects when possible in order to confirm and refine our knowledge of this material. Raman microspectroscopy was the first method applied, being used routinely on all the archaeological objects studied in the project to characterize the lithic materials used by the Amerindians to produce ornamental objects in the Saladoid period. The five objects that could be taken to France were analyzed by a Bruker Senterra Raman microspectrometer equipped with a 532 nm laser, and a 1200-line diffraction grating resulting in a resolution of 3 to 5 cm–1. The objects were analyzed repeatedly and at several locations on each sample with a 50× objective and a nominal laser power of 20 mW in order to confirm the representativeness of the measured signal. Four objects were analyzed at their place of storage in Martinique, Guadeloupe, and Saint Martin during a second phase of the project (table 1) using a portable Raman spectrometer. This Horiba Jobin-Yvon HE532 Raman microspectrometer was equipped with a fixed 920 lines/mm parabolic grating to record the signal in a fixed spectral window from 80 to 3300 cm–1 with a spectral resolution of ~5 cm–1. The analyses were conducted using a long working distance 50× objective with a 532 nm laser whose power was modulated to about 10 mW on the sample.
Infrared spectroscopy was carried out in attenuated total reflection (ATR) mode using a Bruker Alpha spectrometer, measuring absorbance between 400 and 4000 cm–1 with a resolution of 4 cm–1. In all, 48 scans were carried out to obtain a spectrum of sufficient quality, despite the difficulties encountered in positioning the objects and creating sufficient optical contact between the diamond crystal of the ATR and the archaeological artifact without damaging it.
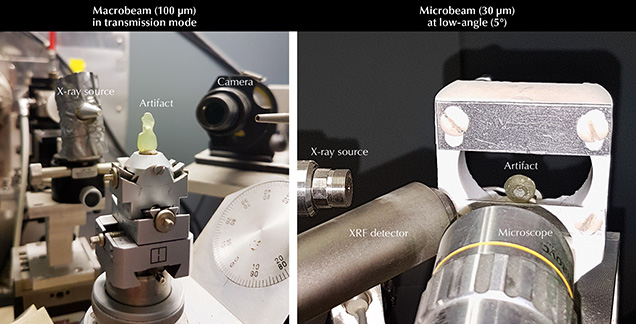
The objects’ mineralogical composition was confirmed by X-ray diffraction. The analyses were carried out using a Rigaku RU200BH X-ray generator, with the Ká line of molybdenum (λ = 0.709 Å). Measurements were performed either in macrobeam (100 μm) in transmission (if the artifact was sufficiently thin) or in microbeam (30 μm) in low-angle (~5°) reflection configuration (figure 3). The diffraction image was recorded and stored on a photostimulated image detector. After scanning, the image was converted to a 2θ diagram using Fit2D software (Hammersley, 2016). Using Bruker’s Diffrac-EVA software, the diagram was then compared to reference databases from the International Centre for Diffraction Data (ICDD) (Gates-Rector and Blanton, 2019).
A portable energy-dispersive X-ray fluorescence (EDXRF) spectrometer allowed qualitative and semi-quantitative characterization. These analyses were carried out using a Bruker Tracer 5i spectrometer, set at 15 kV, 100 μA, 300 s, with a 28 micron aluminum filter to remove the L-lines from the rhodium X-ray tube. Since the artifacts did not systematically cover the whole measurement window, and their thickness was variable, the measured signal of all samples was normalized at 11 keV. Finally, to ensure that certain lines did not come from the measuring device itself, a Teflon pellet was analyzed with the same device, since this material is supposed to contain only elements that cannot be measured by X-ray fluorescence. This Teflon measurement was carried out without a filter so that the L lines of rhodium could be clearly distinguished.
For quantitative analysis, the objects were analyzed by scanning electron microscope coupled with energy-dispersive X-ray spectroscopy (SEM-EDS) in a JEOL IT500HR microscope (FEG source). The SEM images and EDS spectra were acquired without carbon coating in a low-vacuum mode (here 40 Pa). The images were acquired with a backscattered electron detector, while the EDS analysis was performed with two Oxford Ultimax 100 detectors at 20 kV for 30 s, producing spectra with 4.0–4.5 million counts. The chemical quantifications by SEM-EDS in atomic % were converted into atoms per formula unit, normalized to an anionic composition of O10(OH)8 so that they could easily be compared with the chemical formulae known in the literature, both the theoretical formula of the mineral and those measured on natural samples (Bailey and Lister, 1989; Ruiz Cruz and de Galdeano, 2005). The results were also normalized to an anionic composition of O10(OH)2 for comparison with illite. To estimate the volume analyzed, Monte Carlo simulations were conducted in the Casino V2 program (Drouin et al., 2007) using the current (20 kV), matrix composition (Mg, Al, Si, O), and specific gravity (2.65) as parameters.
Two spectrometers were used to obtain the UV-Vis-NIR absorbance spectra of the archaeological objects: a Magilabs GemmoSphere and a PerkinElmer Lambda 1050 equipped with a 3D accessory and an integrating sphere. Measurements with the first instrument provided limited resolution and spectral range (approximately 1.3 nm from 365 to 1000 nm), while the second instrument allowed a resolution of 1 nm in the spectral range from 2300 to 300 nm but required a specific assembly with an accurate placement of the sample upstream of the integrating sphere.
For fluorimetry, we used a Horiba Fluorolog 3. After measuring an emission spectrum with an excitation light set at 420 nm, at a resolution of 1 nm, we obtained an excitation spectrum for the most luminescent object, also at a resolution of 1 nm. In addition, we obtained 3D luminescence spectra, which represent a series of emission spectra (along the x-axis) obtained for a regular sequence of excitation wavelengths (along the y-axis). These 3D spectra thus illustrate the variation of the emission within the range of possible excitation wavelengths.
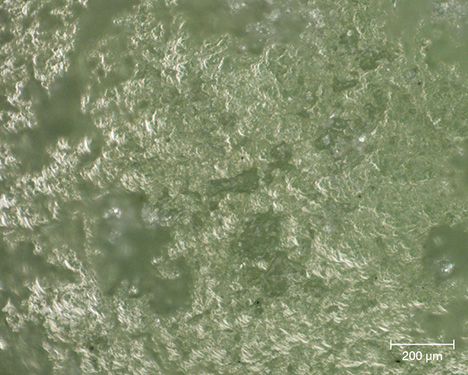
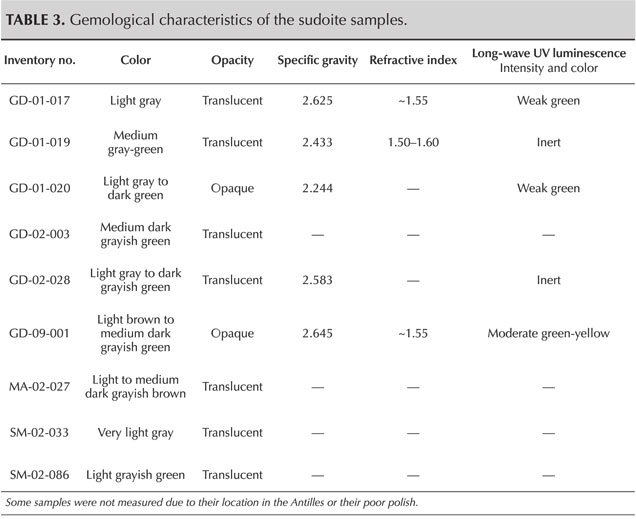
RESULTS
Gemological Properties. Standard gemological observations and measurements were carried out (table 3). Some parameters could be measured only for objects that could be studied outside their place of conservation. The samples here show a certain diversity in terms of opacity and specific gravity. On the other hand, they are all polycrystalline and therefore always appear bright between crossed polarizers; their color is rather homogeneous. Observations revealed a laminated structure, identifiable in some curvatures of the object, where these intersect the main plane of lamination (figure 4). Despite the difficulties in determining the refractive index with a refractometer because of the shape and poor polish of the surface of these archaeological objects, three values between 1.50 and 1.60 were observed, consistent with the values given for chlorite-group minerals, which range between 1.56 and 1.68 (Albee, 1962). Albee (1962) also specifies that a relative decrease in the refractive index is expected when the filling rate of octahedral sites decreases and the magnesium content increases relative to that of iron. In the presence of a di-trioctahedral member of the chlorite group, containing almost only magnesium, it is therefore logical to observe low values in the reported range. No reactions to gemological filters (Chelsea or Hanneman) were noted, and no specific absorption was visible with a handheld spectroscope.
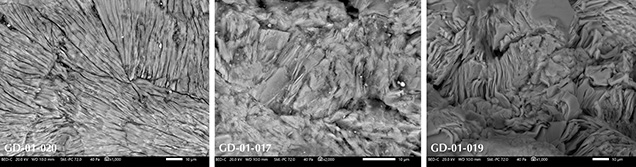
High-Magnification Observation. Backscattered electron (BSE) images acquired by SEM confirm the lamellar structure of the artifacts (figure 5). They also reveal alternating lighter and darker lamellae in some areas of some artifacts (figure 6). This change of grayness level indicates a slight change in chemical composition, with the lighter sheets incorporating heavier elements. Finally, the whole surface of the artifact GD-01-020 shows multidirectional groups of sub-parallel lamellae (figure 5, left) that are not observed in the other samples, where lamellae are poorly organized at a larger scale.
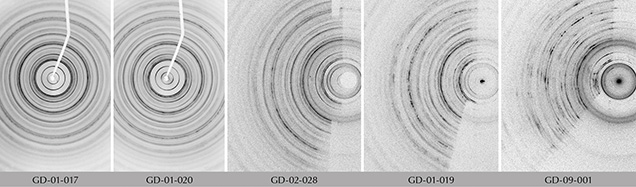
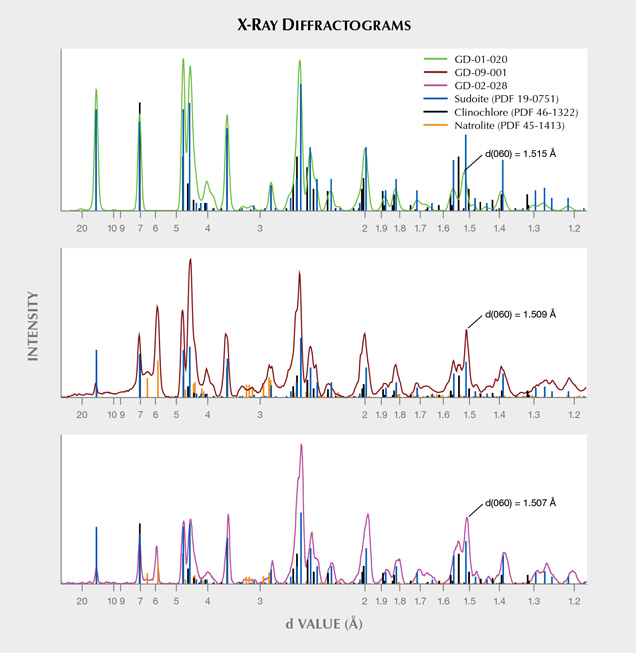
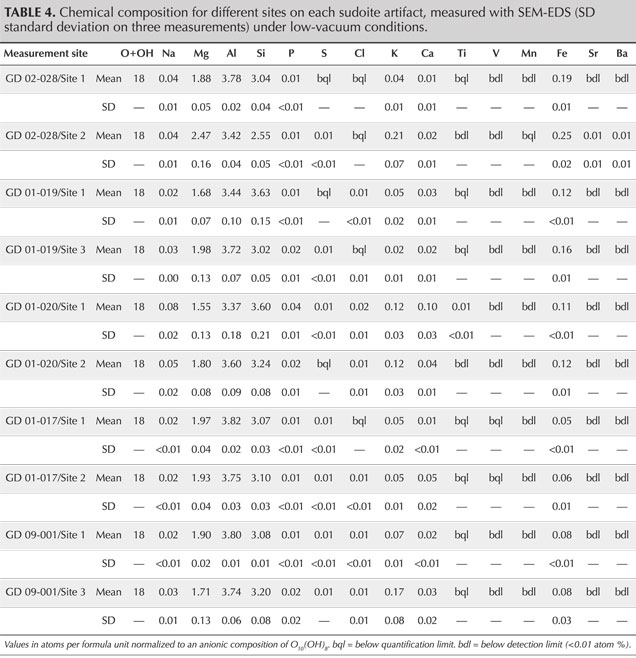
X-Ray Diffraction and Phase Analysis. The diffraction patterns demonstrate the polycrystallinity of the samples and a varying degree of preferential orientation (figure 7). When compared to the PDF2-ICDD crystallographic database, X-ray diffraction clearly confirms the attribution of this material to the chlorite group, and to sudoite in particular (figure 8). The distinction between sudoite and clinochlore is not easy to make by XRD analysis alone, as noted by Bailey and Lister (1989). Three characteristics of the diffraction pattern are mentioned: smaller d(060) values for sudoite (listed as 1.515 Å in table 4 of their work) than clinochlore (1.52–1.56 Å), a d(001) value “slightly smaller” for sudoite, and a “more intense” peak for the (003) reflection (observed near 4.70 Å) in the sudoite pattern. As for our artifacts, we were able to measure d(060) values of 1.518 Å (GD-01-017), 1.509 Å (GD-01-019), 1.515 Å (GD-01-020), 1.507 Å (GD-02-028), and 1.509 Å (GD-09-001). We also observed a very intense (003) reflection in most samples, but our measurements were obtained on massive samples and not powder, so the relative intensity of the peaks cannot be considered representative. Therefore, the data obtained strongly suggest identification of sudoite, and the presence of accessory clinochlore may explain some shoulders and the peak at 1.535 Å (e.g., sample GD-09-001).
This X-ray method probes a larger volume than the very small volume excited by the laser in Raman microspectroscopy. This resulted in the identification of an additional mineral for three of the samples (GD-01-019, GD-02-028, and GD-09-001), namely natrolite Na2(Al2Si3O10)•2H2O, which is a mineral of the zeolite family (figure 8). Natrolite was not identified at the microscopic scale with Raman spectroscopy (figure 10B). The proportion of this mineral, which is highly variable, is difficult to estimate without a Rietveld refinement, which cannot be achieved with our mode of measurement.
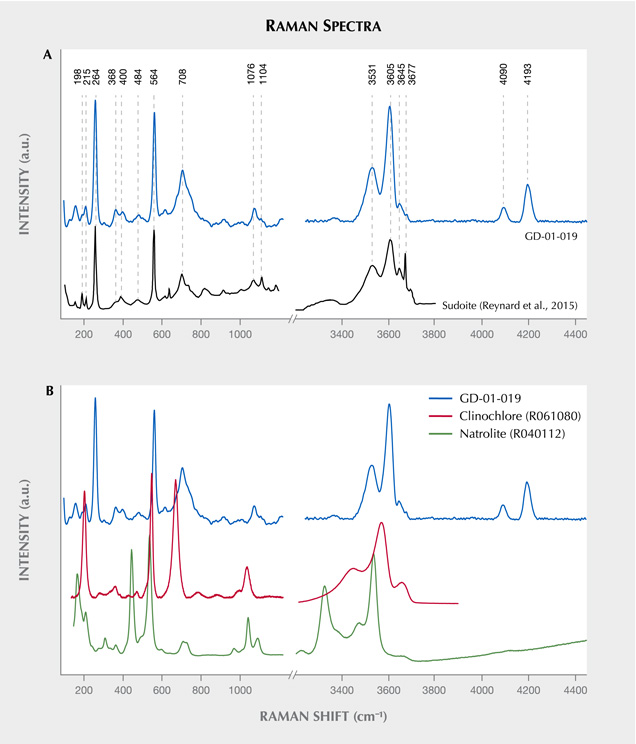
Vibrational Spectroscopy. Raman spectroscopy, the only method applied to all the artifacts presented in this study, illustrates the homogeneity of the composition of these different lapidary objects (figure 9). These results were obtained with desktop equipment on five artifacts. The other four objects were identified as made of the same material, with analysis carried out in museums and other curatorial sites using a portable Raman spectrometer (not shown). A very good agreement can be observed between our results and the only published spectrum for the mineral sudoite (Reynard et al., 2015) (figure 10A), with a correspondence of peak positions not observed with the more common mineral of the chlorite family, clinochlore (figure 10B). Sudoite is positively identified through sharp and intense bands at 264 and 564 cm–1. The bands located between 3300–3600 cm–1 and 3600–3800 cm–1 originate from vibrations of the Al-OH and (Mg, Fe)-OH bonds, respectively (Reynard et al., 2015). Analyzing multiple points on the surface of the archaeological artifacts led to the recognition of rare clinochlore grains, which are probably accessory minerals (as already suggested by XRD characterization), and also of some gypsum, probably the result of pollution on the surface of the samples, which were excavated from coastal sediments.
Infrared spectroscopy (figure 11) confirmed the attribution of the material to the di-trioctahedral chlorite group based on the spectral features in the region of the hydroxyl bond vibrations: The band at 3584 cm–1 originates from vibrations of the (Mg, Fe)-OH bonds, while the presence of two wide bands in the 3300–3500 cm–1 region is interpreted as two different OH-O distances in the interlayer hydroxyl sheets and thus distinctive of the di-trioctahedral structure (Farmer, 1974; Shirozu and Ishida, 1982; Madejová et al., 2017). The characteristic splitting of the Si-O stretching band around 1000 cm–1 in clinochlore, which is absent from our measurements, also supports the identification as sudoite (Madejová et al., 2017). Because of the shape and fragility of the objects, some spectra were difficult to obtain, particularly for the small bead GD-01-020. Comparison with spectra from the literature shows a shift of the main bands in the 400–1200 cm–1 region by approximately 30 cm–1. The sharp band at 830 cm–1, attributed to the (SiAl)OOH bond (Farmer, 1974), however, shows agreement between our experiment and the literature and therefore seems to rule out measurement artifacts. Discrepancies between the data from the literature and our measurements for the archaeological artifacts remain to be explained.
Chemical Characterization. A high degree of chemical homogeneity was observed in EDXRF analysis (figure 12). The major elements are clearly Mg, Al, and Si, consistent with the formula of sudoite, especially taking into account the low efficiency expected for light elements with this method. We also noted the presence of impurities such as P, S, Cl, K, Ca, Ti, V, Fe, and Zn, as well as very low contents of Mn and Ga. The argon (Ar) peak is an artifact related to air in the measured volume.
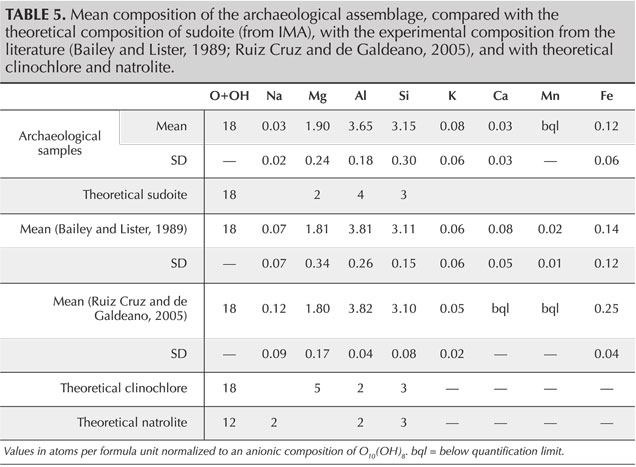
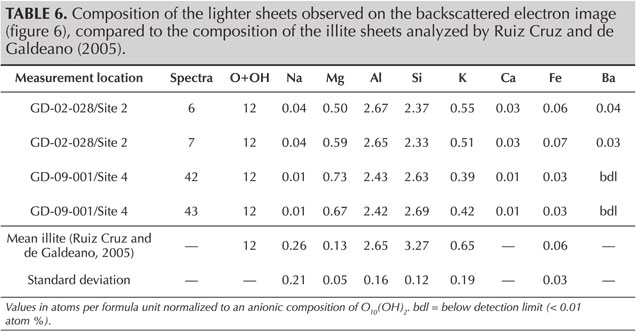
SEM-EDS analyses provide quantification in good agreement with the theoretical composition of sudoite (table 4) and with the published chemical compositions for different sudoite samples from around the world (table 5). Comparative chemical analysis of the light and dark laminae observed in the BSE image shows a higher potassium and/or barium content, correlated with a decrease in magnesium for the light gray sheets (table 6). The balance of charges, modified by potassium (1+) instead of magnesium (2+), is compensated by a decrease in aluminum (3+) in favor of silicon (4+). The composition of the light gray sheets could approach a mixed composition between sudoite and illite, which would be consistent with the possible presence of illite, a mineral often associated with sudoite (Billault et al., 2002; Ruiz Cruz and de Galdeano, 2005). Mixed layers of sudoite with other phyllosilicates have been observed in Transmission electron microscope (TEM) analysis (Ruiz Cruz and de Galdeano, 2005). However, the observation and measurement parameters used during the SEM analysis, optimized for well-focused images of the structure in low-vacuum conditions, did not make it possible to measure only the very thin light gray sheets. Indeed, Monte Carlo simulations indicate that the volume analyzed would be 3–4 μm wide and 3.0–3.5 μm deep.
The natrolite phase, identified by X-ray diffraction in samples GD-09-001, GD-02-028, and GD-01-019, was not identified during the SEM analysis, based on either morphological or chemical data. A few massive grains, lacking any sheet structure, were analyzed, revealing a slightly different chemistry. They were richer in sodium and silicon, with less magnesium and aluminum, but still far from the composition of natrolite (table 5). The analyzed volume given the parameters used (3–4 μm wide and 3.0–3.5 μm deep), being unfortunately larger than the grains themselves (1.0–1.5 μm), prevents us from being conclusive about this. Nevertheless, those grains were very few in number in the artifacts and do not seem to explain the significant diffraction peaks for this mineral phase when present. It is, however, possible that a more in-depth examination of the artifacts exhibiting natrolite by XRD could reveal the distribution and structure of this zeolite mineral.
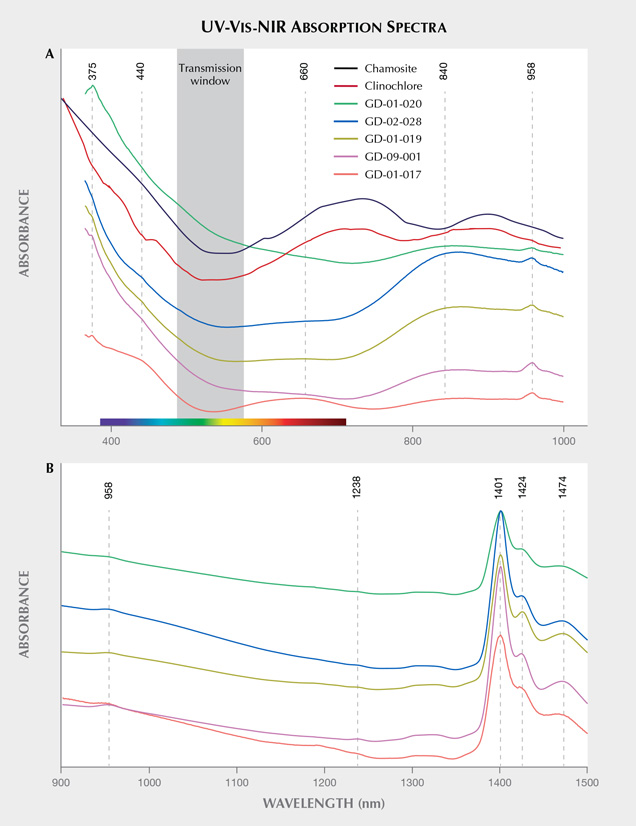
Origin of Color and UV Luminescence. The visible absorption spectra show a continuum of absorption in the violet and the blue; a very wide, shallow transmission band in the green; and absorption rising in the orange and red that is sample-dependent but always greater than in the green (figure 13A). This explains the observed overall green color component in these objects, as described by Fransolet and Bourguignon (1978). The spectra obtained are also quite close to those of other chlorite group minerals analyzed in the literature (Bishop et al., 2008), although it is not clear whether the Fe2+-Fe3+ charge transfer is involved with the bands delimiting the transmission window. The relatively sharp band at 375 nm (26666 cm–1) could be attributed to 4E(D) spin-forbidden transitions of isolated Fe3+ (Krebs and Maisch, 1971; Palanza et al., 2010). The continuum from the UV into the visible region is thus likely related to the O2–-Fe3+ charge transfer, and it explains the brownish yellow color component in some samples. The wide bands around 440 and 660 nm vary together, and may correspond with the position of the bands of V3+ absorption as proposed for vanadium-doped glass by Johnston (1965, figure 1) and Wang et al. (2015). They are indeed the most important for the samples containing the most vanadium (figure 12 and table 4). While the Fe2+-Fe3+ transitions could also have been a candidate for one of these bands, the variation in intensity of the broad absorption bands does not correlate with the concentration levels of this element, and their position is not similar to the band reported in the literature at around 710 nm (14100 cm–1) for chlorite (Mattson and Rossman, 1987). However, Mattson and Rossman (1987) also indicate that the position of absorption bands due to Fe2+-Fe3+ charge transfer is highly variable. It is interesting to note that the value of 710 nm would, on the other hand, correspond very well to wide bands observed in clinochlore and chamosite, two other minerals from the chlorite group. The wide band in the near-infrared, around 830–850 nm and extending well into the visible range, could be attributed to Fe2+ in octahedral position (Spinolo et al., 2007), its intensity correlating with the iron content observed using EDXRF (figure 12) and SEM-EDS (table 4). Thus the transmission window is essentially due to iron: on the UV side essentially by the O2–-Fe3+ charge transfer, and on the red side by Fe2+, with modulation by some V3+.
Finally, water vibrations explain the 958 nm band, as well as absorptions at 1424 and 1474 nm (figure 13B), while O-H bond stretching corresponds to the 1401 nm band (Hunt, 1977; Clark et al., 1990; Clark, 1999; Bishop et al., 2008). These absorption bands could also be interpreted as the water vibrations in the natrolite structure because of their similarity with the spectra obtained by Clark et al. (1990) for this mineral. The absorptions between 1400 and 1500 cm–1 are actually the most intense for samples GD-01-019, GD-02-028, and GD-09-001, which are the three samples exhibiting the most intense natrolite X-ray diffraction peaks.
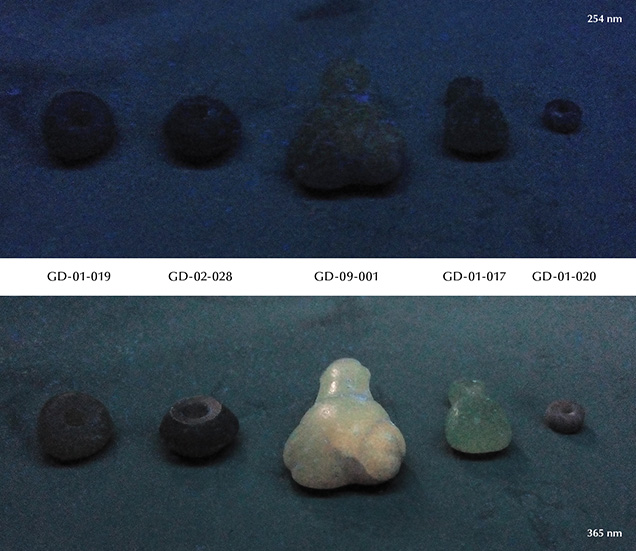
Figure 14 and table 3 group the ultraviolet luminescence observations. Under short-wave UV light, none of the five objects tested show significant luminescence. Under long-wave UV light, three of the five objects tested are homogeneously luminescent, with low to moderate intensity, and show a green to green-yellow color. None show any phosphorescence.
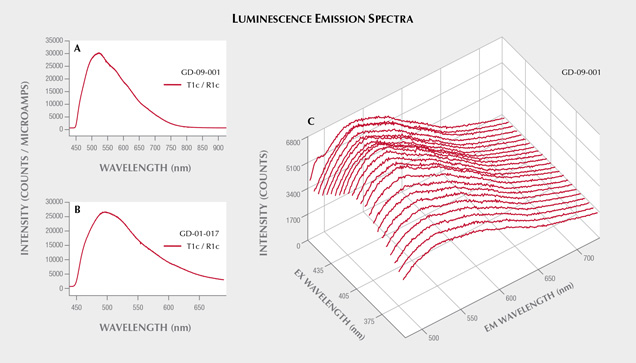
Since the strongest long-wave UV luminescence was observed for samples GD-09-001 and GD-01-017 (figure 14), these two artifacts were also subjected to spectrofluorimetric measurements (figure 15). Note that it is indeed the two samples with the lowest iron content that show this luminescence, as is often the case due to the luminescence quenching character of iron, linked to the O2–-Fe3+ charge transfer (Fritsch and Waychunas, 1994). The emission spectrum with 420 nm excitation is very similar for the two artifacts (figure 15, A and B), suggesting a common origin. The spectrum is asymmetrical, wider on the red side, with probably several components. The maximum is located around 525 nm in the green. Yet the emission covers the entire visible spectrum, inducing a whitish color appearance. The greater sensitivity of the human eye to green wavelengths than blue wavelengths, combined with the color of the object itself that may be seen through the luminescence, results in an observed luminescence color of green-yellow. The 3D spectra obtained on the most luminescent object, GD-09-001, indicate that the luminescence reaches a maximum at 435 nm. Wavelengths lower than 420 nm could not be tested because the measured emission spectrum starts at that point. The same 3D spectra were obtained for GD-01-017 (not shown), and the results were similar. This excitation maximum at 435 nm is somewhat reminiscent of the absorption maximum observed around 440 nm in UV-Vis-NIR spectroscopy, potentially attributed to vanadium. It is also the samples richest in vanadium that show the strongest absorption bands at 440 and 850 nm and exhibit this luminescence, but V3+ is not a common emitter and there are many other possibilities, such as a color center or another trace element ion. Although there are several emissions known for chlorites, the only cause of emission explicitly identified is Cr3+ (Gaft et al., 2005; Czaja et al., 2014).
DISCUSSION
Importance of the Multi-Analytical Approach. The identification of sudoite large enough to be carved deserves certain analytical efforts in order to unambiguously confirm its mineralogical nature. To this end, several types of analysis (physical, chemical, and structural) have been cross-matched. A more common mineral from the chlorite group, whose similar characteristics could lead to confusion with sudoite, is clinochlore. In particular, its X-ray diffractogram is similar to that of sudoite, and it would have been very difficult to formally exclude it, especially given the particular measurement conditions required by archaeological samples. It was possible, though, to rule out clinochlore thanks to the results of vibrational and UV-Vis spectroscopy as well as elemental analyses that confirmed a composition matching that of sudoite. Raman spectra and the sheet structure could have led to a misidentification as pyrophyllite or muscovite on the basis of some main Raman bands, but the XRD, FTIR, and chemical analyses precluded this possible confusion. Clinochlore and natrolite are present as accessory minerals in some samples. The former is identified very rarely by Raman spectroscopy, as only a few points on the surface of the artifacts show clinochlore, and in XRD. The latter is present in the XRD pattern of some artifacts.
As a material used to produce lapidary artifacts, one could also be interested in its characterization by more classic gemological methods. Unfortunately, the polycrystalline nature, limited polish, and shape and size of the objects, as well as the absence of a non-archaeological reference material, have limited the possibilities of these approaches (e.g., the rather variable specific gravity and the difficulty of measuring the refractive index). The green color could be related to the sum of Fe3+-, Fe2+-, and V3+-related absorption bands, leaving mostly a shallow transmission window in the green. The UV luminescence of the material is documented for two samples, but its cause remains unclear.
Geological Origin. Sudoite has previously been identified in various geological contexts. The main type of rock in which sudoite is found has undergone low-temperature/high-pressure metamorphism in a subduction context (Fransolet and Bourguignon, 1978; Goffé et al., 1988; Ruiz Cruz and de Galdeano, 2005). The stability field of sudoite is between 240 and 380°C, and between 4 and 8 kbar (Goffé et al., 1988). In this context, it can come from an intermediate stage of metamorphism between a dickite assemblage and a chlorite + paragonite + chloritoid assemblage, in which it is found associated with pyrophyllite, which is not the case for our samples (Ruiz Cruz and de Galdeano, 2005). It may also result from retrograde metamorphism, as proposed in Oman, with a decrease in pressure synchronous with a slight increase in temperature (Goffé et al., 1988). Sudoite can form from kaolinite in the last stages of diagenesis, above 200°C (Daniels et al., 1990), and it is also encountered in a hydrothermal context, at temperatures higher than 200°C (Beaufort et al., 2015).
Sudoite is usually found in the form of fine clay (Billault et al., 2002; Lauf, 2010). Small rosettes up to 1 mm in diameter can form (Beaufort et al., 2015), as can scaly films (Gaines et al., 1997, cited in Lauf, 2010). Only one occurrence of centimeter-sized sudoite large enough to be carved could be found in the literature (figure 15 in Lauf, 2010), underscoring the rarity of such samples. Beaufort (2015) writes:
The only type of hydrothermal system in which sudoite has been reported in large amounts and in large volumes of altered rocks are unconformity-related uranium deposits (Percival and Kodama, 1989; Billault et al., 2002; Beaufort et al., 2005).
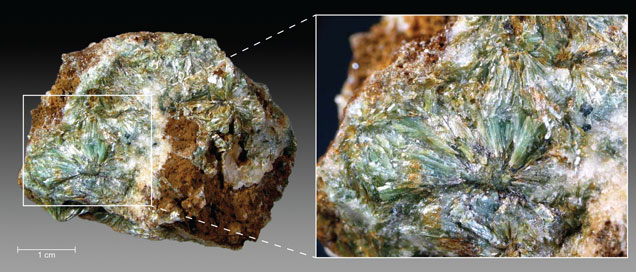
He more recently confirmed this to the authors: “With respect to ... sudoite occurrences in the Paleoproterozoic basins of Canada and Australia, the concept of massive can only be applied on a microscopic scale, because on a macroscopic scale this mineral is always associated with other clay minerals such as illite and kaolinite or dickite. To my knowledge, there is no gem sudoite in this type of geological context” (D. Beaufort, pers. comm., 2019). Rare larger-volume samples consist of agglomerates of radial structures, with color and transparency close to those observed in the artifacts we studied (figure 16). They strongly resemble one studied and shown in, unfortunately, a poor-quality photograph (figure 2 in Jige et al., 2003). These agglomerates are also reminiscent of the structures observed by electron microscopy on sample GD-01-020 (figure 5).
Provenance of the Antillean Archaeological Raw Material. The tectonic context of the Caribbean presents high-pressure/low-temperature (HP/LT) metamorphic rocks, especially due to the numerous subduction zones (Garcia-Casco et al., 2011; see figure 17). The suture of these subduction zones produced ophiolite (Garcia-Casco et al., 2006; Lewis et al., 2006), which can be the site of sudoite crystallization, as is the case in Oman (Goffé et al., 1988). Metapelites (metamorphic rocks resulting from the transformation of fine clay-rich sediments from the alteration of continental crust) subjected to a metamorphism of blueschist facies of low intensity can produce rocks containing magnesian chlorites and in particular sudoite (Goffé et al., 1988; Schreyer, 1988; Theye et al., 1992). Rocks formed under HP/LT conditions in the blueschist facies, common in the Caribbean and consistent with the presence of natrolite in some samples, would thus be an excellent candidate for the provenance of this material. It should also be noted that the morphology of the sudoite we observed under the electron microscope (figures 13 and 14) differs greatly from Canadian hydrothermal crystallizations (figure 2 in Billault et al., 2002). This criterion may also indicate that HP/LT metamorphism is more likely to be involved in the crystallization of sudoite than hydrothermal metamorphism.
The presence of sudoite in the Lesser Antilles adds to an existing set of converging clues in the mineralogy of gem materials used in Amerindian lapidary craftsmanship that indicate “long-distance” transport of the raw material. These raw materials, for which no precise source has been clearly stated, originate from rocks that do not naturally exist in the Lesser Antilles and must therefore come from the South American continent and/or the Greater Antilles (Cody, 1993; Murphy et al., 2000; Queffelec et al., 2018, 2020). Previous studies of hard rocks such as jadeitite, used for the manufacture of a polished ax, have already highlighted such trade networks from the Greater Antilles to the Lesser Antilles (e.g., Harlow et al., 2006; Garcia-Casco et al., 2013; Schertl et al., 2019). The greenish translucent material sudoite could therefore have been part of the batch of exotic colored rocks brought to the Lesser Antilles as part of the pan-Caribbean trade network described for this period (e.g., Rodriguez, 1993; Hofman et al., 2007; Rodriguez Ramos, 2010; Laffoon et al., 2014). It should also be noted that a source of nephrite, a material frequently found in collections of ornamental objects from the same period, was recently discovered on the northern coast of Colombia (Acevedo Gómez et al., 2018), not that far from the Panamanian or Costa Rican ophiolites.
Of the nine artifacts identified as sudoite, eight are of centimeter-sized dimension, consistent with most of the other lithic beads and pendants of Amerindian cultures (e.g., Murphy et al., 2000; Queffelec et al., 2018, 2020; Falci et al., 2020). Such small pieces require only limited sizes of mineralization. Only one pendant (GD-02-003) has a size of several centimeters. Even though such large volumes of raw material may be rare, they must have been available at the outcrop itself to be exploited by the Amerindians, since it is unlikely that this kind of sheet silicate does occur in secondary deposits given its fragility. These outcrops must be of very limited dimensions, which may explain the absence of known localities so far.
All of these objects were found on three islands of the French Lesser Antilles distributed along the Caribbean arc. Sudoite has been identified in every archaeological site that delivered dozens of lapidary artifacts known for these three islands. This underscores the possibility that this newly identified lapidary material could also be found among the archaeological collections of other islands distributed in the same cultural network, if detailed analytical studies were carried out. Identifying this material in archaeological collections will require analytical techniques such as Raman spectroscopy, which is not systematically used by archaeologists, especially in the Caribbean.
CONCLUSIONS
Sudoite was unambiguously identified using a multi-analytical strategy combining methods both structural (Raman and infrared spectroscopy, X-ray diffraction, SEM) and chemical (XRF and EDS). This magnesium-rich di-trioctahedral sheet silicate from the chlorite group is confirmed to be the raw material used by the Amerindians of the Early Ceramic period in the Lesser Antilles to create the nine personal ornaments in this study. It is also the first time this material has been identified as being used for lapidary craft from any period, and the first time it has been described in sizes suitable for carving. The origin of the color and the long-wave UV luminescence properties have also been explored by means of UV-Vis-NIR spectroscopy and spectrofluorimetry. The greenish color is related to the presence of iron (both Fe2+ and Fe3+), with a possible contribution from V3+ that would narrow the transmission window for some artifacts. The cause of the UV luminescence remains unclear.
Such a rare material is of interest for the archaeologist, since it may offer valuable information on trade networks during the period under consideration. Sudoite is one of the green rocks used in Amerindian lapidary craftsmanship in the Lesser Antilles, possibly originating from the Greater Antilles, or Central or South America. Based on metamorphic geology, it is possible to consider the origin of sudoite in the ophiolitic formations located at the sutures of the collisions between the Caribbean plate and the North American and Cocos plates. These particular zones are therefore found mainly in northern Cuba, Panama, and Costa Rica, but finding outcrops of this material will prove very difficult due to their limited size in primary deposits and their limited dispersal in secondary deposits. As it would be particularly important to specify the archaeological distribution of the sudoite raw material and its geographical origin, this calls for further detailed analytical studies of objects from the archaeological sites, both in the Antilles and on the nearby continent, and for detailed field prospecting in the areas that might have generated this material.